Analyzing the Spectral Region in Science and Technology
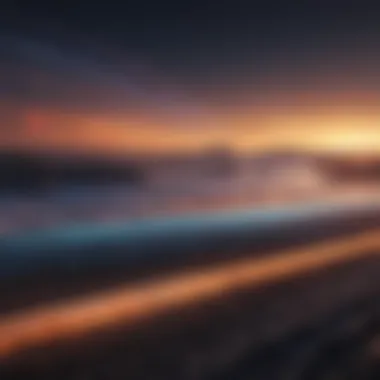
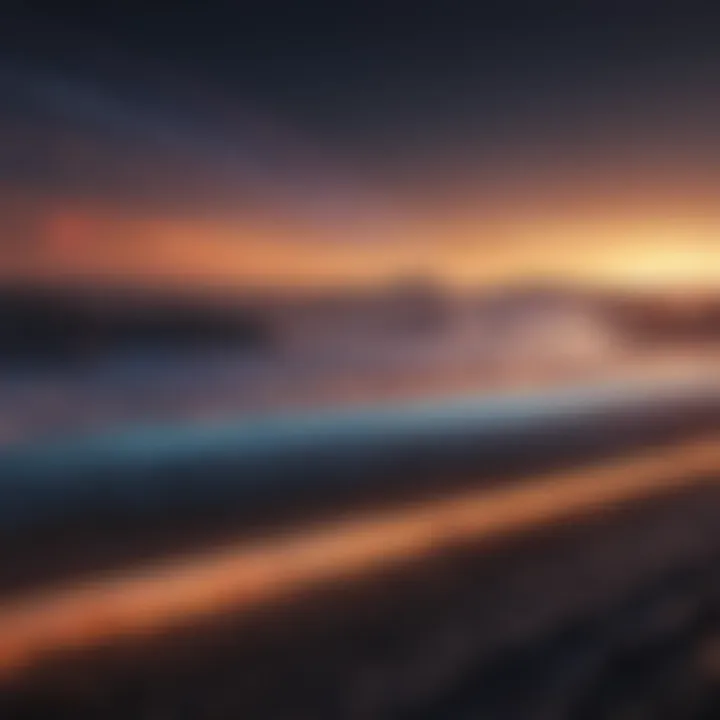
Intro
In the vast landscape of science, the spectral region plays a pivotal role in unearthing the mysteries of matter and its interactions with electromagnetic radiation. This overarching theme resonates across multiple disciplines, particularly physics and chemistry, where the exploration of different wavelengths reveals profound insights into the nature of materials and energy. From understanding atomic structures to developing cutting-edge technology, the significance of these spectral regions cannot be overstated.
This analysis aims to provide an in-depth look into the nuances of spectral measurement and classification, as well as the methodologies employed in research. By unpacking the various aspects of spectral regions, we pave the way for a more comprehensive grasp of their implications in both scientific discovery and technological innovation.
Methodologies
Description of Research Techniques
When delving into spectral regions, researchers utilize a variety of techniques to comprehend the complex interactions of light and matter. At the heart of this investigation are methods such as spectroscopy and photometry. Spectroscopy, for instance, allows scientists to dissect light into its constituent wavelengths, revolutionizing our ability to analyze materials. Techniques like infrared and ultraviolet-visible spectroscopy are quintessential for examining chemical compounds, revealing how different substances respond to various wavelengths.
Moreover, advancements in nonlinear optics have introduced innovative techniques. For example, harmonic generation enables probing molecular dynamics with unprecedented temporal resolution. Techniques like these ensure scientists are not merely spectators but active participants in unveiling the layers of molecular behavior.
Tools and Technologies Used
The backbone of spectral analysis often rests on an array of specialized instruments. These tools enable researchers to conduct accurate measurements and interpretations. Some significant instruments include:
- Spectrophotometers: Essential for measuring the intensity of light as a function of wavelength. They are indispensable in fields like environmental science and biomedical research.
- Mass Spectrometers: Particularly useful in identifying chemical compounds through their mass-to-charge ratios. This technology plays a crucial role in fields ranging from pharmaceuticals to forensics.
- Fluorescence Microscopes: These allow researchers to observe biological structures at a molecular level, providing insights into processes like cell signaling and disease mechanisms.
Each tool provides unique insights that, when combined, offer a richer perspective of the spectral region's intricacies.
Discussion
Comparison with Previous Research
Reflecting on past studies allows us to contextualize current findings within the broader scientific narrative. For example, the evolution of spectroscopic techniques from basic prism methods to modern laser-induced breakdown spectroscopy illustrates significant strides in precision and application. Previous research predominantly focused on individual components of spectroscopy, whereas contemporary studies emphasize integrative approaches that combine multiple spectral techniques for a holistic understanding of materials.
Theoretical Implications
The implications of spectral region analysis extend well beyond the lab bench. The interactions of light with matter lead to profound theoretical implications. Understanding these interactions not only aids in material science but leads to advancements in quantum mechanics and photonics.
"The exploration of spectral regions is akin to opening a window into a complex world. The more we understand about these wavelengths, the clearer our understanding becomes of the universe itself."
Researchers are continually working to refine theoretical frameworks that explain these interactions in more nuanced terms, paving the way for future innovations in technology and science.
Prolusion to the Spectral Region
The spectral region is a cornerstone concept in understanding the intricate dance between light and matter. Its significance extends across various scientific fields, particularly in physics and chemistry, where the nuances of electromagnetic radiation play a pivotal role in research and technological advancements. By diving into the spectral region, we can unlock insights into a multitude of applications such as environmental monitoring, medical diagnostics, and materials science.
Understanding this region allows scientists and researchers to decode the signals emitted or absorbed by different substances. Shadows, colors, and patterns—in essence, the often invisible interactions between light and matter—hold keys to broader scientific inquiries and practical applications. This article offers a comprehensive exploration of these interactions, methodologies for measurement, and classifications inherent to various spectral regions, shedding light on their importance in both fundamental and applied sciences.
Defining the Spectral Region
The spectral region refers to specific ranges of wavelengths of electromagnetic radiation. Each region carries distinct characteristics, influencing how different materials respond to it. In essence, the spectral region serves as a framework that categorizes the electromagnetic spectrum based on wavelength and frequency.
When we refer to electromagnetic radiation, we are addressing not only visible light, which the human eye can see, but also other forms of radiation that exist beyond our perception. While visible light has wavelengths ranging from approximately 400 to 700 nanometers, other spectral regions extend far beyond, encompassing radio waves at one end and gamma rays at the other. Thus, defining the spectral region is crucial as it lays the groundwork for understanding how various forms of radiation interact with matter.
For scientific purposes, accurately defining these regions is a necessity. The scientific community generally divides them into categories based on their wavelength and energy levels, from the low-energy radio waves to the high-energy gamma rays. Such definitions pave the way for specialized research and technological applications that stem from these interactions.
Historical Context
To grasp the depth of the spectral region, one must consider its historical emergence. The study of light and its properties can be traced back to ancient civilizations, but significant strides began in the 17th century with figures like Isaac Newton, who famously used a prism to disperse light into its component colors. This revelation initiated a quest for understanding wavelengths and the properties associated with various colors.
As science advanced, the 19th century marked a turning point with the development of spectroscopy. Scientists such as Robert Bunsen and Gustav Kirchhoff highlighted the role that light plays in identifying substances, paving the way for practical applications in chemistry and physics. Their work led to the establishment of what we now recognize as atomic spectroscopy—the analysis of light emitted or absorbed by atoms and molecules.
The understanding of the spectral region continued to evolve through the 20th century, incorporating modern advancements in technology. The introduction of lasers and increasingly sophisticated detectors has transformed how we study the interactions of electromagnetic radiation with matter. Inmates in fields such as astrophysics and environmental science have since utilized these advancements to glean insights from spectral data.
In summary, defining the spectral region contextually and historically underscores not only its intrinsic value but also its centrality in various scientific applications. As we move forward, the dialogue around these regions continues to develop, signaling a future ripe with possibilities for discovery and innovation.
Fundamental Concepts
Understanding the fundamental concepts of the spectral region is crucial for various scientific fields. These concepts form the backbone of how different wavelengths of electromagnetic radiation interact with matter and play a significant role in numerous applications, from medical diagnostics to environmental monitoring. Recognizing these principles not only fosters a better understanding of spectroscopy but also enhances the ability to analyze and interpret data effectively. With advancements in technology and interdisciplinary collaboration, a firm grasp of these concepts can lead to innovative solutions and discoveries.
Electromagnetic Spectrum Overview
The electromagnetic spectrum is a continuum of electromagnetic radiation that varies in wavelength and frequency. It ranges from very long radio waves to the very short gamma rays, encapsulating various types of radiation that we encounter in our daily lives. Each section of the spectrum serves a unique purpose and has distinct characteristics.
- Radio Waves: Used in communications; essential for television and radio broadcasts.
- Microwaves: Effective for heating food and in radar technology.
- Infrared: These waves are utilized in thermal imaging and remote controls.
- Visible Light: The only part of the spectrum humans can see, it plays a key role in vision and photosynthesis.
- Ultraviolet Light: Important for sterilization and can cause sunburn.
- X-rays: Commonly used in medical imaging to view inside the body.
- Gamma Rays: Produced by radioactive elements, these have applications in cancer treatment and are fundamental in understanding cosmic phenomena.
Each segment of the spectrum interacts with matter in unique ways, offering scientists valuable insights into atomic and molecular structures.
Wavelength and Frequency Relationships
The relationship between wavelength and frequency is more than just numbers; it illuminates the very nature of light itself. Wavelength is the distance between successive peaks of a wave, while frequency is how often these peaks pass a given point per second. The two are inversely related, meaning that as wavelength increases, frequency decreases, and vice versa. This relationship is expressed by the simple equation:
[ c = \lambda \cdot f ]\
Where:
- c = speed of light (approximately 299,792,458 meters per second)
- λ (lambda) = wavelength
- f = frequency
Understanding this interplay is essential in fields like telecommunications, where specific frequencies are used for different communication technologies. For example, longer wavelengths (lower frequencies) are better suited for broadcasting over large distances, while shorter wavelengths (higher frequencies) can carry more information but have a limited range.
Energy Levels and Quantum Transitions
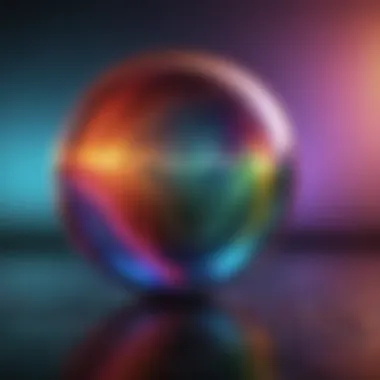
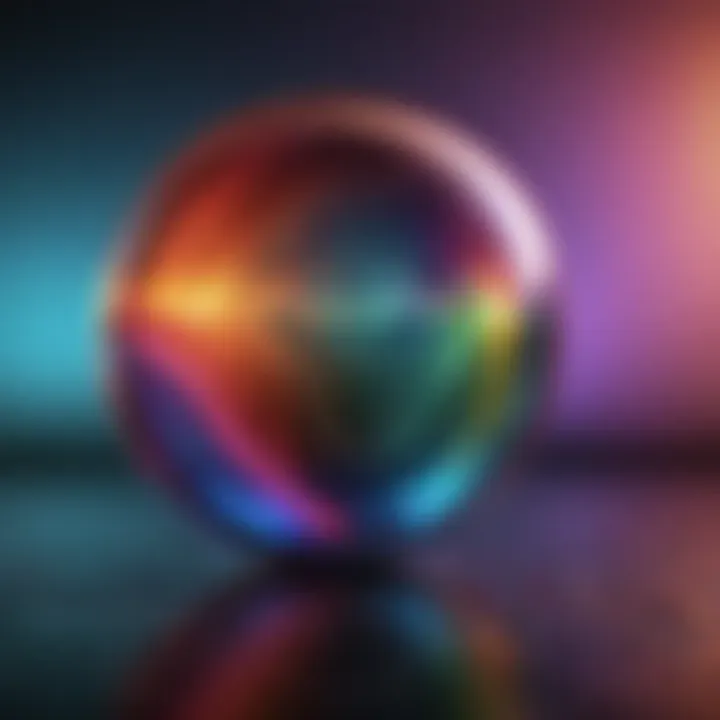
At the core of the spectral analysis lies quantum mechanics, specifically the concept of energy levels and quantum transitions among atoms and molecules. Each atom has discrete energy levels where electrons can reside. When energy is added to an atom—whether through electromagnetic radiation or thermal energy—electrons can move between these levels.
This transition can lead to:
- Absorption: When an electron moves to a higher energy level, absorbing light in the process.
- Emission: When an electron falls back to a lower energy state, releasing energy in the form of light.
The wavelengths of light emitted or absorbed during these transitions are characteristic of specific substances, acting like a fingerprint. This is what makes spectroscopy such a powerful tool in identifying materials and understanding chemical properties.
"Spectroscopy serves as the bridge connecting the microscopic world with macroscopic observations."
In summary, mastering these fundamental concepts provides researchers and practitioners a solid foundation to explore the spectral region's nuances and its vast applications in modern science.
Classification of Spectral Regions
Understanding the classification of spectral regions is fundamental in grasping how different wavelengths of electromagnetic radiation interact with matter. Each spectral region has its own properties, uses, and methods of measurement, which are crucial for applications in various scientific fields. This classification offers clarity, guiding researchers and practitioners in selecting the appropriate methods for analysis and experimentation.
The classification also enhances our comprehension of the electromagnetic spectrum, fostering advancements in technology, from communication systems relying on radio waves to medical imaging that utilizes X-rays. Moreover, recognizing how these regions interact with matter undoubtedly opens doors to novel research opportunities, fostering innovation across multiple disciplines.
Radio Waves
Radio waves, which encompass the longest wavelengths in the electromagnetic spectrum, play a critical role in communication systems. Their ability to travel long distances with minimal interference makes them indispensable for broadcasting and telecommunications. For instance, the FM and AM radio formats operate within this range, utilizing modulated signals to transmit audio across vast areas.
The principle of frequency modulation, in particular, allows radio waves to carry more information, enhancing the clarity of sound. Scientists are constantly exploring radio waves’ interactions with various materials, leading to advancements in fields like remote sensing and even astrophysics, where they study cosmic phenomena.
Microwaves
Microwaves follow radio waves in the spectrum and have shorter wavelengths ranging from one meter to one millimeter. They are widely known for their use in cooking appliances, as they heat food by agitating water molecules. More than just kitchen appliances, microwaves facilitate various technologies, including radar and satellite communications.
In the field of materials science, microwaves enhance the study of chemical reactions and can assist in monitoring material stability. Their capacity for penetration through certain substances makes them valuable in medical applications, such as MRI technologies that examine the bodily tissues without ionizing radiation.
Infrared Region
The infrared region, lying beyond visible light, is characterized by wavelengths that are longer than visible light but shorter than microwaves. Infrared radiation is key to heat detection and thermal imaging, making it valuable in numerous applications including night vision technology and weather forecasting.
In environmental science, infrared spectroscopy allows researchers to study molecular structures and interactions, thus monitoring environmental changes. It’s used in analyzing greenhouse gases and tracking pollution, demonstrating its pivotal role in safeguarding our environment.
Visible Light Spectrum
Spanning the narrow range visible to the human eye, this spectrum forms the crux of daily experience. Each color we perceive corresponds to specific wavelengths, stretching from violet at about 400 nanometers to red at around 700 nanometers. Light interaction with matter within this region is fascinating; it plays an essential role in photography, vision, and plant photosynthesis.
Moreover, the visible spectrum enables various scientific measurements and instruments, such as spectrophotometers, which assess the concentration of substances by measuring light absorption. Thus, the visible light spectrum is much more than mere colors; it's a gateway for understanding life itself.
Ultraviolet Region
Ultraviolet (UV) light extends just beyond the visible light spectrum and possesses higher energy, which allows it to break molecular bonds. UV radiation is crucial in various fields, from sterilization processes in healthcare to in-depth studies in chemistry where it helps analyze compounds through UV-Vis spectroscopy.
However, UV light must be approached with caution due to its potential harmful effects. Overexposure can lead to skin damage and other health concerns, so researchers are interested in both the beneficial and detrimental aspects of UV radiation.
X-rays
X-rays can penetrate soft tissues but are absorbed by denser materials like bones, which gives them a vital role in medical diagnostics. The ability to produce clear images of internal structures with minimal invasiveness revolutionized modern healthcare. The Ct scans and X-ray imaging technologies rely on the properties inherent in X-rays.
In the realm of materials science, X-ray crystallography has helped scientists understand the atomic structure of various substances. By studying the scattering patterns of X-rays, researchers gain insights that facilitate the design of new materials at the molecular level.
Gamma Rays
The highest frequency and greatest energy are found in gamma rays, which arise from nuclear reactions and certain types of radioactive decay. Their penetrative capabilities have made them fundamental in medical therapies, especially in cancer treatment where targeted radiation can destroy malignant cells.
Moreover, gamma rays contribute to astrophysics, where they provide information about cosmic events, such as supernovae and black hole activity. Exploring gamma rays continues to broaden our understanding of the universe, challenging researchers to deepen their knowledge of fundamental physics.
"Understanding spectral regions not only empowers us to harness their potential but also provides insight into the underlying principles that govern our physical world."
In summary, the classification of spectral regions serves as a cornerstone of various scientific inquiries, with each category offering unique insights and vast applications. The exploration of these spectral fields enriches our comprehension and shapes future innovations.
Interaction of Light with Matter
The relationship between light and matter is a crucial aspect of many scientific disciplines. Understanding how light interacts with different substances allows us to explore numerous phenomena—from basic scientific principles to advanced technological applications. These interactions can be categorized mainly into three processes: absorption, emission, and scattering. Each of these interactions offers valuable insights into the properties of materials and the composition of chemical substances.
When we look at absorption spectroscopy, we begin to see how materials absorb specific wavelengths of light. This process not only makes it possible to identify substances based on their absorption characteristics but also provides information about molecular structure and concentration. Such techniques are crucial in fields ranging from environmental monitoring to quality control in manufacturing.
Emission spectroscopy, on the other hand, reveals how materials emit light in response to energy changes. This emission can tell us about excited states of electrons and is instrumental in identifying elemental compositions and finding traces of materials. It's fascinating how the light released during certain transitions can offer a unique fingerprint for various elements, which has profound implications in fields like astronomy and forensic science.
Scattering phenomena, too, deserve mention, as they reflect the influence of light interacting with particles. The way light scatters can inform researchers about particle size, shape, and even the composition of substances, making this aspect particularly valuable in atmospheric studies and materials science.
Understanding the nuances of these interactions provides critical knowledge applicable to both theoretical research and practical applications. The insights gathered from studying light’s interaction with matter help guide advancements in technology, environmental assessment, and medical diagnostics.
"The ability to comprehend and manipulate the interaction of light with matter stands as a cornerstone of modern scientific inquiry."
Absorption Spectroscopy
Absorption spectroscopy elucidates how materials absorb electromagnetic radiation. This technique is predominantly utilized to analyze various substances, enabling the identification and quantification of chemical species in diverse environments. When light passes through a sample, specific wavelengths are absorbed by the material, which can be attributed to electronic transitions of molecules or atoms.
In practical applications, this method is invaluable. For example, in chemical analysis, it helps identify the presence of specific compounds in a mixture by measuring the intensity of light absorbed at certain wavelengths. The Beer-Lambert Law illustrates this relationship, positing that absorbance is directly proportional to concentration—the higher the concentration of the absorbing species, the greater the absorbance of light.
The significance of absorption spectroscopy extends beyond the laboratory, as well. It is employed in environmental monitoring to detect hazardous substances like pollutants in air or water. By measuring how specific wavelengths are absorbed, scientists can identify the concentration of toxins, providing an essential tool for public health and safety.
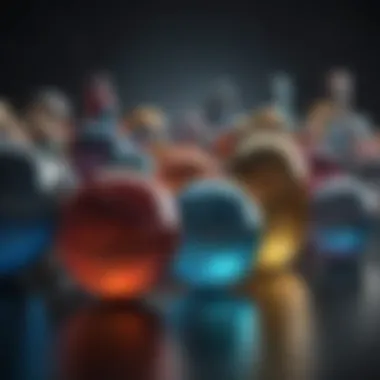
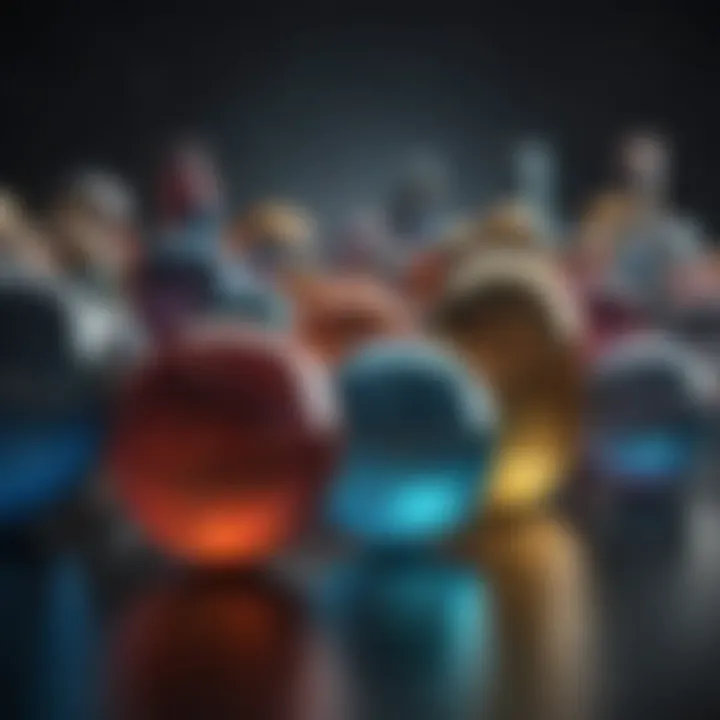
Emission Spectroscopy
Emission spectroscopy involves the analysis of light emitted by substances after they have absorbed energy. When an atom or molecule gains enough energy, its electrons are excited to higher energy levels. Once they return to their ground state, they release this energy as light. This light can be examined to reveal details about the material's elemental composition.
One notable technique in this realm is Flame Emission Spectroscopy, often used in detecting metals in samples. When a sample is introduced to a flame, the heat excites the electrons, causing them to emit characteristic light of specific wavelengths. This process enables us not only to identify the presence of various elements but also to measure their concentrations accurately.
Emission spectroscopy finds its usage spanning many fields: from astrophysics, where emission spectra of distant stars provide information about their compositions and temperatures, to biological sciences, where researchers may identify compounds in cellular structures. As such, it helps depict a vivid picture of both natural and artificial phenomena.
Scattering Phenomena
Scattering phenomena describe how light changes direction when it encounters particles. This interaction can provide vital information about the particles themselves, including their size, shape, and composition.
A prominent example is Raleigh scattering, which explains why the sky appears blue. Shorter wavelengths of light (like blue) scatter more than longer wavelengths (like red), shedding light on atmospheric conditions. This concept transcends mere observation and extends into various practical applications.
In medicine, for example, dynamic light scattering is utilized to analyze the size distribution of small particles, which is essential in drug formulation. Here, knowing how drugs disperse can indicate their effectiveness and bioavailability. In environmental science, scattering techniques can be applied to monitor aerosols, providing insight into air quality and climatic changes.
Understanding scattering provides depth to our comprehension of both natural phenomena and technical applications. By investigating how light interacts with matter in different contexts, we can reveal underlying principles that guide numerous scientific endeavors.
Measurement Techniques in Spectroscopy
In the fascinating realm of spectroscopy, measurement techniques are the backbone that allows scientists to decode the richness of interactions between light and matter. Each method presents its unique strengths and considers various factors, ultimately contributing to our understanding of the spectral region. Understanding these techniques is crucial as they serve not only academic inquiry but practical applications across industries.
Spectrophotometry
Spectrophotometry stands out as one of the foundational tools in spectroscopy. This technique measures the amount of light absorbed by a sample at different wavelengths. The basic premise relies on Beer's Law, which links absorbance to concentration, making it a powerful method for quantitative analysis.
- Key Importance:
- Simple and cost-effective, making it widely accessible.
- Extremely useful for characterizing the concentration of substances in solutions, ranging from biological samples to environmental samples.
But spectrophotometry isn't without its challenges. The accuracy of the measurements can be affected by:
- The presence of particulates or bubbles in a solution.
- The choice of solvent, which may absorb some wavelengths itself.
- Calibration issues that may arise with aging equipment or user neglect.
With care and standardization, these obstacles can often be managed, and the results can provide clarity in the intricate world of chemical analysis.
Fourier Transform Infrared Spectroscopy
Fourier Transform Infrared Spectroscopy (FTIR) exemplifies another stellar technique in the spectroscopy toolkit. FTIR captures the infrared light spectra of a substance, offering insights into molecular vibrations and functional groups present in a sample. By effectively decoding the complex data via Fourier Transform, this method translates intricate signals into actionable information.
- Benefits:
- Non-destructive, preserving the integrity of samples, which is crucial in fields like forensics or art conservation.
- Rapid data processing means that results can be achieved quickly, allowing researchers to pivot in their investigations as necessary.
Nevertheless, FTIR carries its own set of considerations. The need for careful sample preparation and the potential for water vapor interference in the spectra necessitates meticulous attention to detail. Researchers must acclimate themselves to these nuances, fostering proficiency in obtaining accurate, reliable readings.
Raman Spectroscopy
Raman Spectroscopy brings yet another layer of complexity and depth to the table. By harnessing inelastic scattering of monochromatic light, the technique reveals vibrational, rotational, and other low-frequency modes in a system. This method is especially favored for its capability to analyze samples without requiring extensive preparation.
- Key Applications:
- Particularly effective for studying heterogeneous mixtures and identifying molecular composition.
- Can be used in real-time monitoring and often in situ technology, which is invaluable in various research scenarios such as material science and pharmaceutical development.
As with other methodologies, Raman Spectroscopy isn’t exempt from issues. The random nature of the scattering process may lead to low signal intensity, making a robust signal amplification technique or appropriate background subtraction integral for reliable results.
"The true power of spectroscopic measurement techniques lies in their ability to uncover the hidden nuances of molecular interactions, often leading to groundbreaking discoveries in science and technology."
Applications of Spectral Analysis
Spectral analysis plays a pivotal role across various fields, providing researchers the tools to decode the hidden messages within light. The importance of this topic cannot be overstated—it is not just an academic exercise but a vibrant area of exploration with real-world implications.
The applications stretch from the monitoring of environmental conditions to ensuring safety and efficacy in medical diagnostics. Each use case showcases the fundamental link between wavelengths and matter interactions, highlighting their relevance to both theoretical and applied sciences. With a wealth of benefits, spectral analysis aids in problem-solving, enhances image resolution in materials research, and aids in faster, more precise medical evaluations. It’s no wonder that this area garners interest among students, researchers, and professionals alike.
"The link between spectral analysis and real-world applications is not just about data; it's about understanding and improving the world we live in."
Environmental Monitoring
The role of spectral analysis in environmental monitoring is crucial. By employing techniques such as remote sensing, scientists can evaluate changes in land use, assess the health of ecosystems, and monitor atmospheric pollution. The analysis of light reflected from the Earth's surface allows for the identification of different materials, vegetation types, and even health-issues like chlorophyll concentration in plants.
Some specific applications include:
- Air Quality Assessment: Using spectrometers to detect and quantify gaseous pollutants like nitrogen dioxide and sulfur dioxide.
- Water Quality Monitoring: Analyzing water samples to check for contaminants through absorption spectroscopy, which identifies harmful substances based on how they absorb light at particular wavelengths.
Thus, the strategies behind environmental monitoring hinge upon spectral analysis, making it indispensable for sustainable practices and ecological studies.
Material Science
In material science, spectral analysis serves as a lens into the atomic and molecular structure of substances. Researchers use it to understand material properties, behaviors under different conditions, and potential applications in technology. For instance, Fourier Transform Infrared Spectroscopy (FTIR) and Raman Spectroscopy lend insights into molecular vibrations and chemical composition, leading to innovations in various sectors.
Key benefits in this field include:
- Characterization of Materials: Determining the composition and phase of substances, which is crucial in developing new materials.
- Quality Control: Ensuring materials meet required specifications through continuous monitoring.
- Failure Analysis: Investigating failed components using spectral methods to diagnose root causes and prevent future issues.
The impact of spectral analysis in material science is profound; it not only catalyzes advancements in research but also has significant implications in manufacturing and industrial applications.
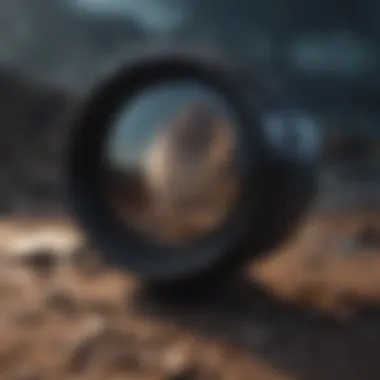
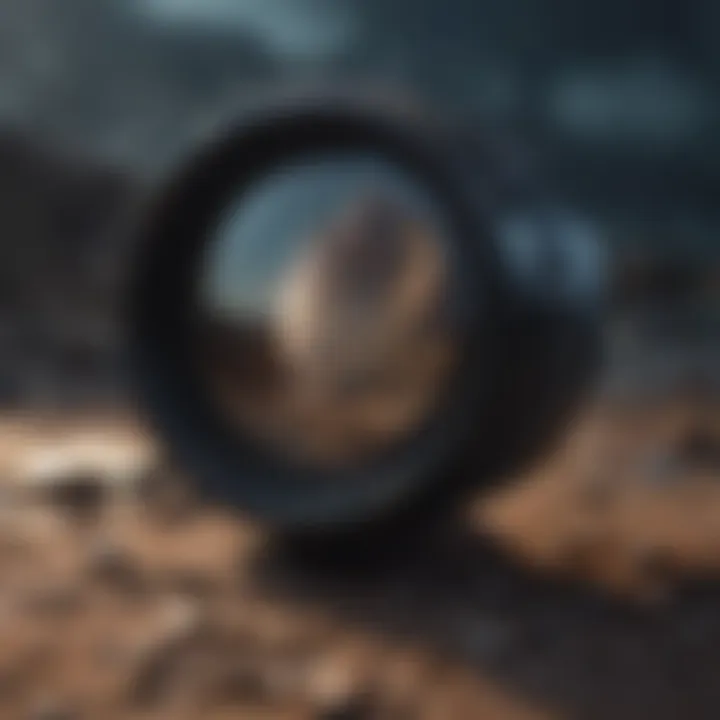
Medical Diagnostics
The application of spectral analysis in medical diagnostics has revolutionized the way diseases are detected and monitored. Techniques such as absorbance spectroscopy and fluorescence spectroscopy show potential for non-invasive assessments of bodily fluids, offering faster diagnostics with high accuracy.
In medical contexts, some points of interest are:
- Detection of Biomarkers: Spectroscopy can identify specific molecules that indicate the presence of a disease, helping in early diagnosis of conditions like cancer.
- Therapeutic Monitoring: Regular analysis of blood samples through spectral methods can indicate how well a treatment is working, allowing for timely adjustments.
- Imaging Technology: Spectral imaging techniques help create high-resolution images of tissues and organs, supporting doctors in making better-informed decisions.
In sum, spectral analysis in medical diagnostics illustrates how understanding light-matter interactions can have profound effects on human health, paving the way for innovations in health monitoring and treatment effectiveness.
Challenges and Limitations
In the realm of spectroscopy, recognizing the challenges and limitations is just as crucial as embracing its capabilities. The field has advanced significantly, yet hurdles remain that can impact the accuracy and reliability of spectral measurements. Understanding these challenges illuminates why ongoing research and improvements in techniques are vital. It’s not just about what we can see with the instruments; knowing the limitations helps us appreciate the full picture.
Instrumental Challenges
Instrumental challenges in spectroscopy often come down to the very equipment that researchers rely on. Instruments must be finely calibrated and maintained, and any downtime or failure can compromise data collection. For example, consider the situation with high-resolution spectroscopy devices. If the optics or sensors aren't functioning optimally, the resulting spectra can become distorted. Such artifacts can lead to misinterpretation, affecting everything from atmospheric studies to material characterization.
- Precision Variability: The precision and accuracy of measurements can differ across various instruments. This may lead to inconsistent results, making it difficult to establish standard benchmarks.
- Maintenance Requirements: Annual or even bi-annual maintenance is essential. If a researcher cannot afford this or if the downtime impacts crucial experiments, the research could face significant delays.
Moreover, technological obsolescence represents a lurking challenge within instrumentation. As new methods emerge, older technologies may fall short. It requires constant investment and upgrading, where funds and resources might not always be available. Knowing these instrumental challenges can shape better frameworks for future research pathways.
Calibration Issues
Calibrating spectroscopic equipment accurately is akin to setting the stage for a performance. If the initial setup is flawed, everything that follows is likely to be off-key. Calibration issues typically arise when the care in preparing the instrument doesn’t match the complexity of the sample being analyzed.
- Standard Reference Materials: The use of various reference materials for calibration can introduce discrepancies. It’s important to routinely check these standards to ensure they hold up under scrutiny.
- Environmental Factors: Temperature variations, dust, and humidity levels can affect calibration. For instance, an uncalibrated machine in a humid environment may read spectroscopic lines broader than they are, leading to misinterpretation of chemical compositions or structures.
Another layer of complexity is that certain measurements require recalibration as conditions change. This adds to the workload and can become cumbersome for researchers.
Interference Factors
Finally, interference factors during spectral analysis can muddy the waters of clarity. External influences, whether from the environment or the materials themselves, can affect the purity of the spectroscopic data.
- Chemical Interference: In a mixed sample, overlapping absorptions or emissions can confuse analysis. For instance, if researchers are studying a sample containing both water and organic compounds at similar wavelengths, it becomes painstaking to distinguish their signals.
- Physical Interference: There’s also physical interference to consider. If ambient light infiltrates the spectroscopic measurement environment, it may skew results and lead to erroneous conclusions.
"Identifying and addressing these interference factors is essential for accurate spectral analysis and reliable data interpretation."
Monitoring these challenges can guide researchers in developing more robust methodologies. The deeper understanding of these limitations not only enhances the integrity of scientific inquiry but also encourages innovation in solving persistent spectroscopy hurdles.
Future Trends in Spectroscopy
As we glance into the crystal ball of spectroscopic techniques, it’s clear that future trends indicate a period of significant innovation and evolution. The importance of this topic cannot be overstated, as advancements in spectroscopic methods hold the potential to leapfrog current analytical capabilities. Innovations in hardware, enhancements in data analysis algorithms, and the convergence of fields are key aspects driving this transformation, benefiting various scientific disciplines and industries.
Advancements in Technology
In recent years, we have witnessed remarkable strides in technology that are reshaping the landscape of spectroscopy. Miniaturization of devices, such as the development of portable spectrometers, is a game-changer. Instead of being confined to laboratory settings, spectroscopy can now be conducted in the field, providing real-time data collection. For instance, handheld spectrometers enable environmental monitoring with instant analysis of pollutants in air and water.
Moreover, the integration of artificial intelligence into spectroscopic analysis has opened doors to rapid data interpretation. Machine learning algorithms can identify patterns that traditional methods might overlook. For instance, using AI in mass spectrometry can significantly increase accuracy in identifying complex mixtures, from pharmaceutical metabolites to contaminants in food.
Another exciting development is the advent of quantum optics in spectroscopy. Techniques that utilize quantum entanglement promise to enhance resolution and sensitivity far beyond the reach of classical techniques. Such advancements might not just improve signal-to-noise ratios but could also expand our understanding of interactions at a quantum level.
Research Directions
The ongoing research in spectroscopy takes multiple routes, responding to current challenges and societal needs. One promising area is the search for novel materials and methods that improve detection capabilities. For example, nanomaterials like graphene are being investigated for their potential to enhance sensor performance. The unique properties of these materials could lead to breakthroughs in detecting trace elements at exceedingly low concentrations.
Furthermore, researchers are focusing on combining spectroscopic techniques with other analytical methods for a multifaceted approach. The fusion of NIR spectroscopy with imaging techniques can provide both spatial and compositional data, facilitating more detailed analysis in fields such as biology and medicine.
On the frontiers of research, scientists are diving deeper into the spectroscopic analysis of complex systems, like biological samples. Moving into the realm of single-cell analysis brings about exciting possibilities. The future could very well see techniques that allow researchers to unravel the nuanced biochemical behaviors of individual cells, shaping our understanding of diseases and leading to tailored medical treatments.
Interdisciplinary Approaches
Interdisciplinary collaboration stands as a beacon of future spectroscopic advancements. The merging of physics, chemistry, biology, and even informatics is becoming increasingly commonplace. By pooling expertise from various disciplines, scientists can tackle complex issues and develop innovative solutions that a single field might struggle to address alone.
For instance, partnerships between biologists and chemists have accelerated the development of advanced imaging techniques. These collaborations enable a deeper understanding of biological processes through spectroscopic methods, facilitating breakthroughs in drug discovery and diagnostics.
Moreover, incorporating insights from computer science can refine data processing in spectroscopy. With vast data pools being generated, efficient data mining techniques are paramount. The development of algorithms tailored for spectroscopic data analysis is paving the way for more profound insights and faster conclusions.
"Innovation distinguishes between a leader and a follower." – Steve Jobs
By embracing these trends, we are not just witnessing the future of spectroscopy; we are actively shaping it.
Culmination
The conclusion of an article like this one holds weighty significance. Summing up the complex web of interactions between electromagnetic radiation and matter necessitates clarity and precision. By synthesizing the key takeaways, this section becomes a lens through which readers can grasp the broader implications of the spectral region in their respective fields.
Here are the salient elements addressed in this article:
- Importance of Understanding Spectral Regions: To appreciate the nuances of materials and their interactions with light is fundamental in research across various scientific disciplines.
- Interdisciplinary Applications: The diverse applications of spectral analysis—from medical diagnostics to environmental monitoring—illustrate its relevance and utility.
- Future Directions: Highlighting ongoing research fosters a sense of curiosity and encourages the community to delve deeper into this expansive field.
Shall we summarize the essence? The spectral region is not just a scientific curiosity; it serves as a bridge connecting multiple disciplines. As technologies advance, the methodologies for spectral analysis will likely evolve, potentially leading to breakthroughs that we can't even fathom today. Thus, it’s vital to maintain focus on this area of research—doing so allows science to progress in ever-expanding horizons.
Summary of Key Points
- Electromagnetic Spectrum: Understanding the electromagnetic spectrum is crucial for various applications in science and industry. The interactions between light and matter shape everything from technological advancements to natural phenomena.
- Measurement Techniques: Modern spectroscopy techniques allow us to dissect the spectral region accurately, enabling a deeper understanding of materials at both macro and micro levels.
- Classification of Regions: The classification of spectral regions enables researchers to target specific wavelengths for investigation, making it an invaluable organizational framework in spectroscopy.
- Challenges: Recognition of the challenges facing spectroscopy, such as instrument calibration and interference factors, provides a clearer roadmap for potential solutions moving forward.
The Importance of Ongoing Research
Ongoing research in the spectral field is paramount— it’s akin to opening a Pandora's box of possibilities. The quest for knowledge in this area is endless, with new technologies and methodologies constantly emerging. Every advancement holds the chance to improve our understanding of the universe through the lens of light.
- Traditional methods are being challenged by newer, more accurate technologies, enhancing capability in fields ranging from environmental science to material characterization.
- By fostering interdisciplinary collaboration, scientists can enrich their understanding and improve application strategies across various domains.
- Furthermore, ongoing research can lead to novel applications, such as innovative medical diagnostic tools or new materials with enhanced properties.
Indeed, the importance of continued exploration in spectral analysis cannot be understated. Each breakthrough enhances not just theoretical knowledge, but practical applications that can significantly alter our understanding of the world around us. As we stand at the threshold of new discoveries, the journey through the spectral region will undoubtedly guide us toward a more advanced scientific narrative.