Understanding X-Ray Diffraction Testing: Essentials
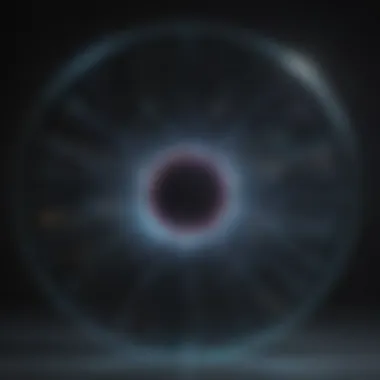
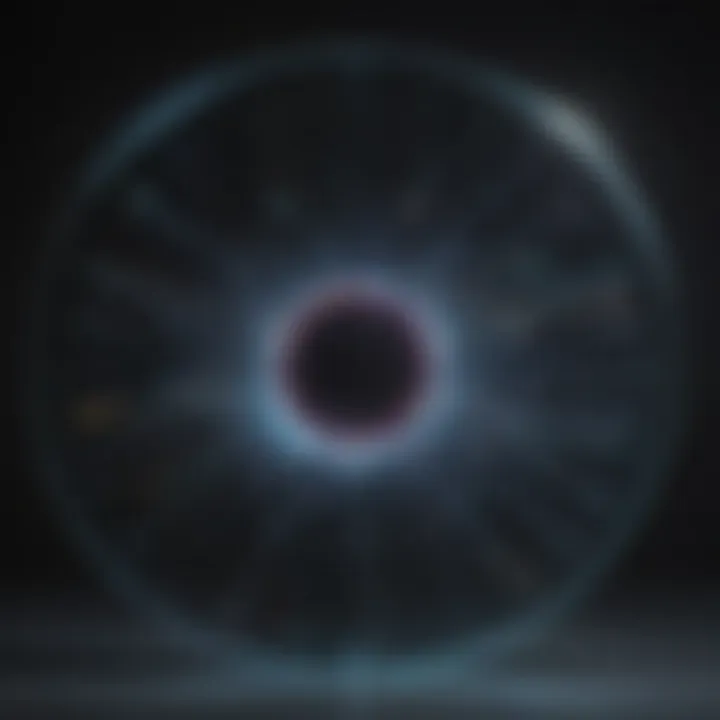
Intro
X-ray diffraction (XRD) is a vital technique in the field of materials characterization. It provides insights into the crystallographic structure of materials and is instrumental across various scientific disciplines, including chemistry, physics, and geology. This article delves into the methodologies and practical applications of XRD testing and explores its relevance in current research trends and advancements.
Understanding the principles behind XRD is essential for anyone involved in materials science or related fields. This comprehensive overview will cover not only the theoretical aspects but also practical applications and future implications of XRD in scientific inquiry. It aims to serve students, researchers, educators, and professionals, enhancing their understanding of this crucial analytical method.
Methodologies
Description of Research Techniques
X-ray diffraction involves directing X-rays at a material and analyzing how they scatter to provide information about the atomic structure. The basic principle follows Bragg's Law, which relates the angles at which X-rays are diffracted to the spacing between the atomic planes in a crystal. This relationship allows scientists to deduce the crystallographic arrangement of atoms in the tested material.
In a typical XRD experiment, a sample is placed in the path of an X-ray beam. As the beam interacts with the sample, it is diffracted at specific angles. These angles are measured and plotted as a diffraction pattern, which serves as a fingerprint for the material's crystalline structure.
Tools and Technologies Used
The execution of XRD tests relies heavily on specialized equipment. Key components of XRD systems include:
- X-ray Source: Commonly a copper or molybdenum target source, the choice of source can impact resolution and the type of samples analyzed.
- Goniometer: This device allows controlled rotation of the sample and detector. Precise positioning is critical for accurate measurements.
- Detector: This device captures the diffracted rays and converts them into a signal that can be analyzed. Popular types include silicon strip detectors and photon counting systems.
Some advanced XRD techniques may utilize synchrotron radiation, which provides high-intensity beams for more detailed studies. Furthermore, combined methods like XRD-SEM (Scanning Electron Microscopy) can yield complementary information about the sample.
Discussion
Comparison with Previous Research
XRD has evolved significantly over the past few decades. Initially, the technique was limited to bulk materials and single crystal samples. Recent developments have expanded its applicability to nanomaterials and complex multi-phase systems. Researchers have concurrently explored novel structures or components like thin films, layers, and composites, greatly enhancing the versatility of the method.
Theoretical Implications
The theoretical background of XRD is underpinned by solid-state physics, allowing for the interpretation of crystal symmetry and lattice parameters. Advancements in computational modeling have also taken XRD to new heights, enabling simulations that predict diffraction patterns, reducing the need for extensive experimental work. This interplay between theory and practice is critical, offering promising avenues for future research.
"XRD remains a cornerstone technique for material analysis, but new innovations continually reshape our understanding of its potential."
Prolusion to X-Ray Diffraction Testing
X-Ray Diffraction (XRD) testing has emerged as a crucial analytical technique in various scientific fields. Understanding its foundations is essential for students, researchers, and professionals involved in material characterization. The significance of XRD extends beyond mere analysis; it plays a vital role in advancing knowledge and applications in materials science, chemistry, geology, and even biology.
One key element of XRD is its ability to reveal intricate details about the internal structure of crystalline materials. This is critical for determining phase identification and quantifying the composition of samples. Furthermore, XRD provides insights into the crystallography of materials, aiding in the development of new materials and improving existing ones.
Another important consideration is the versatility of XRD. It can be applied to powder samples, single crystals, and even thin films. This adaptability makes XRD a powerful tool in both academic research and industrial applications, allowing for a wide range of analyses across different disciplines.
In this section, they will delve into the definitional framework and historical milestones of XRD, setting the stage for a deeper examination of its principles, methodologies, and applications.
Definition and Importance of XRD
X-Ray Diffraction is a technique used to study the structure of crystalline materials by directing X-rays at a sample and analyzing the resulting diffraction pattern. The pattern formed provides critical information regarding the arrangement of atoms within the crystalline lattice, lending insight into phase transitions, crystal structures, and even stress and strain in materials.
The importance of XRD lies in its non-destructive nature, allowing for analysis without altering or damaging the sample. This is particularly valuable in scenarios where preserving the integrity of the material is paramount. Moreover, XRD's ability to provide precise quantitative analysis of phase compositions is unmatched, making it a cornerstone of research and quality control in various industries.
Historical Context of XRD Development
The development of X-Ray Diffraction can be traced back to the early 20th century. Max von Laue first proposed the idea in 1912, leading to significant advancements in crystallographic research. Following his initial experimentation, the utilization of X-rays to investigate crystal structures expanded rapidly.
In subsequent decades, scientists such as William Henry Bragg and William Lawrence Bragg refined the method and formulated Bragg's Law, providing a mathematical framework to interpret diffraction patterns. Their contributions marked a pivotal moment in the field, enabling more systematic studies of crystalline materials.
From its inception, XRD has evolved considerably, integrating advancements in technology that enhance both speed and accuracy. This historical trajectory illustrates the transformative role that XRD has played in scientific research, with applications now spanning a remarkable array of fields.
In summary, the introduction to X-Ray Diffraction Testing establishes a fundamental understanding of its importance and relevance in various domains. By exploring its definition, significance, and historical development, the foundation is laid for a thorough examination of the principles, methodologies, and applications that follow.
Principles of X-Ray Diffraction
The principles of X-Ray Diffraction (XRD) are fundamental to understanding its application and relevance in various scientific fields. By examining the way X-rays interact with crystalline materials, one can uncover essential information about the structure and properties of these materials. The importance of this topic lies not only in the basic mechanics of diffraction but also in the broader implications for material analysis, research, and technological development.
Basic Principles of Diffraction
X-ray diffraction works on the principle of wave interference. When a beam of X-rays strikes a crystalline material, it interacts with the electron clouds surrounding the atoms in the crystal structure. This interaction follows specific geometric patterns determined by the arrangement of atoms within the crystal lattice. As X-rays are scattered in various directions, constructive and destructive interference occurs, leading to a unique diffraction pattern that can be measured and analyzed.
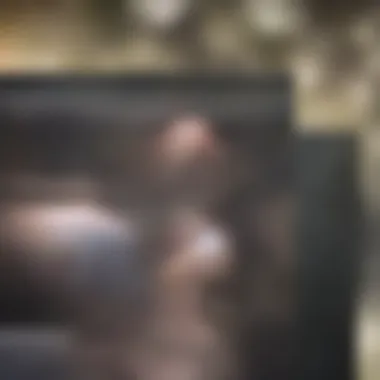
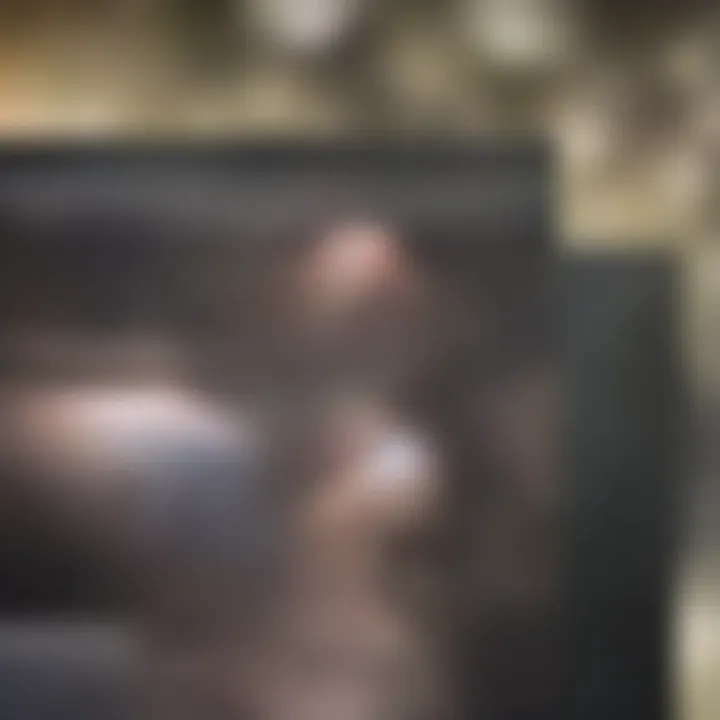
To facilitate a deeper understanding:
- Wavelength and Crystal Spacing: The wavelength of the X-rays must be comparable to the distance between the atoms in the crystal. This condition ensures that diffraction can effectively occur.
- Reciprocal Lattice: The concept of reciprocal space is essential in diffracting patterns. The arrangement of points in reciprocal space gives insight into the symmetry and dimensions of the crystal structure.
These principles form the foundation upon which various XRD techniques are built, directly influencing their specificity and efficacy.
Bragg's Law and Its Significance
Bragg's Law is a cornerstone of X-ray diffraction, providing a quantitative relationship that allows for the calculation of the angles at which X-rays are diffracted by a crystal. This law states:
nλ = 2d sin(θ)
where:
n = order of reflection,
λ = wavelength of X-rays,
d = distance between atomic planes,
θ = angle of incidence.
The significance of Bragg's Law cannot be overstated. It serves multiple purposes in the realm of XRD:
- Structural Analysis: By applying Bragg's Law, researchers can determine the spacing between atomic planes. This information is crucial for characterizing the structure of materials.
- Identification of Phases: Different materials will yield unique diffraction patterns. By deciphering these patterns, scientists can identify and quantify various phases within a sample.
- Quality Assessment: The law can also be applied to assess the quality of crystalline materials. A well-defined peak in a diffraction pattern indicates a well-ordered structure, while broad peaks suggest disorder.
Understanding these principles and Bragg's Law provides a solid basis for interpreting XRD data, ultimately aiding in material characterization and research efficiency.
Methodologies in XRD Testing
The methodologies used in X-Ray Diffraction (XRD) testing are pivotal for the successful application of this analytical technique. These methodologies ensure that the results obtained are both reliable and reproducible. This section elaborates on the key methodologies involved in XRD, focusing on specific elements, advantages, and considerations.
Sample Preparation Techniques
Proper sample preparation is crucial in XRD testing as it directly impacts the quality of the diffraction data obtained. The sample must be homogeneous and appropriately sized to minimize errors in the diffraction pattern. Here are several key strategies for effective sample preparation:
- Grinding and Homogenization: Samples, particularly powders, must be ground to a fine particle size. This reduces the chances of preferred orientation and enhances statistical representativeness.
- Pelletizing: For certain applications, it may be beneficial to compress powders into pellets. This method helps form a dense, uniform layer and could improve diffraction quality.
- Thin Film Techniques: When working with thin films, special attention is needed. These samples often require substrate considerations and growth parameters that affect crystallinity.
- Mounting and Orientation: Properly mounting samples in the XRD apparatus ensures correct alignment with the X-ray beam. Incorrect mounting may lead to misleading results. A consistent method of sample placement contributes to reliable data collection.
Instrumental Setup and Calibration
Once the sample is prepared, setting up the XRD instrument correctly is the next critical step. The instrumental setup and calibration process guarantees accurate and reproducible results.
- Instrument Configuration: Understanding the components of the XRD machine is crucial. This includes knowing the role of the X-ray tube, goniometer, and detector in the collection of data. Correct alignment ensures that the incident beam and detector angles facilitate effective data capture.
- Calibration Procedures: Regular calibration of the instrument is necessary. Calibration typically involves the use of standard reference materials with known diffraction patterns to verify that the system produces accurate results. Without proper calibration, misinterpretations can arise.
- Software Utilization: Most modern XRD systems come with sophisticated software for data analysis. Ensuring that this software is up to date is important for both data collection and interpretation.
"An accurate sample preparation and instrumentation setup process significantly reduces challenges in data interpretation."
In summary, the methodologies in XRD testing are foundational in extracting reliable data from this complex technique. From sample preparation to instrumental calibration, each step contributes to the integrity of the analysis.
The effectiveness of these methodologies influences the breadth and depth of applications, ranging from material science to biological studies.
Types of XRD Techniques
Understanding the different types of X-Ray Diffraction (XRD) techniques is crucial for effectively applying this analytical method in various scientific fields. Each technique offers unique advantages and specific capabilities, serving different purposes based on the material or compound being analyzed. These techniques can guide researchers in selecting the appropriate method for their particular analysis, ensuring accurate and reliable results.
Powder XRD Analysis
Powder XRD analysis is one of the most widely used forms of XRD due to its versatility and effectiveness. It is particularly beneficial in materials science for characterizing crystalline materials. The sample is crushed into a fine powder, which allows for the averaging of crystallographic orientations, leading to more comprehensive data. This approach is advantageous when dealing with polycrystalline materials, as it provides a more significant dataset and improved statistical reliability.
Key benefits of Powder XRD include:
- Ability to identify phase compositions through comparison with standard reference patterns.
- Useful in determining lattice parameters and grain sizes, which are essential in material characterization.
- Suitable for analyzing complex mixtures, as it can resolve different phases present in a sample.
Single Crystal XRD Analysis
Single crystal XRD analysis is specifically designed for examining individual crystalline structures rather than powders. This technique provides in-depth information about the arrangement of atoms within a crystal lattice, enabling precise measurements of bond lengths, angles, and overall atomic positions. Researchers often turn to this method when they need to understand the detailed structural information of small single crystals, such as new compounds or biomolecules.
A distinct advantage of single crystal XRD is its ability to derive more complex structural information from single crystals, which can be critical for applications in chemistry and biology. However, growing suitable crystals can be challenging, and only a small number of materials can be successfully analyzed using this technique.
High-Resolution XRD
High-resolution XRD is an advanced technique that enables extremely precise measurements of diffraction patterns. It is often used in studies of thin films, layered materials, and complex nanostructures. The increased resolution allows researchers to detect subtle changes in the crystal lattice caused by various factors such as strain or defects.
Considerations for using High-Resolution XRD include:
- The technique generally requires specialized equipment and careful sample preparation.
- It can provide insights into microstructural features, which is valuable in fields like materials science and solid-state physics.
- High-resolution measurements can aid in studying structural phase transitions and other dynamic processes.
"Choosing the right XRD technique can significantly influence the quality and reliability of your analytical results."
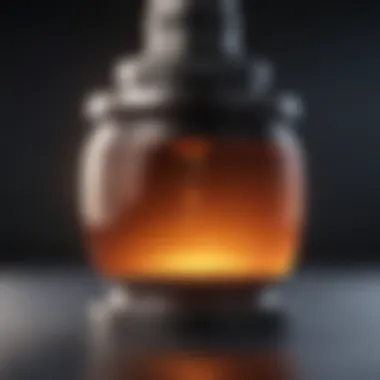
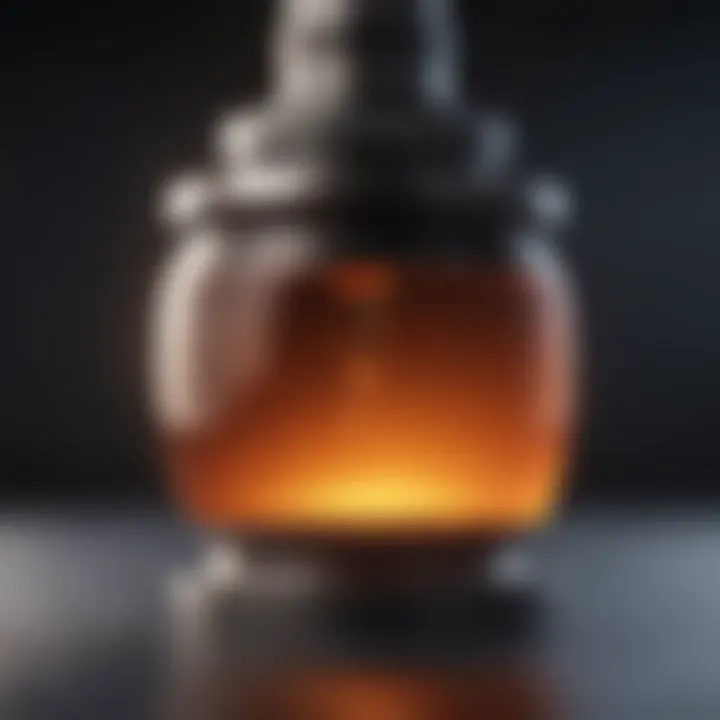
For further reading on X-ray diffraction techniques, you may visit Wikipedia.
Applications of XRD Testing
The applications of X-Ray Diffraction (XRD) testing extend across various scientific domains, establishing it as a critical tool in material analysis. This versatility is essential for both academic and industrial sectors. Recognizing the specific elements and benefits of XRD applications aids researchers and professionals in leveraging this technique effectively. The accuracy and precision of XRD have profound implications for material characterization, facilitating advancements in numerous fields and fostering innovation.
Materials Science Applications
In materials science, XRD plays a vital role in determining the structural properties of new materials. It helps in the identification of phase compositions, which is crucial when developing alloys or ceramics. For instance, understanding crystal structure can lead to enhancements in material performance, including strength, ductility, and thermal properties.
Moreover, XRD is beneficial in monitoring material degradation and phase transformations. This is particularly important in the aerospace and automotive industries, where material reliability is paramount. Researchers often rely on powder XRD to evaluate the crystallinity of polymers or composite materials. By analyzing diffraction patterns, they can assess how additives or processing conditions affect material characteristics.
Role in Chemistry Research
XRD also bears significance in chemistry research, particularly in the study of molecular structures. Chemists utilize XRD to elucidate the arrangement of atoms within a given substance, which aids in understanding chemical reactions and interactions. For example, in coordination chemistry, XRD provides insights into the geometry around metal centers in compounds, influencing their reactivity.
Additionally, XRD can be applied to investigate complex mixtures, such as catalytic materials. By identifying phases present in these systems, researchers can optimize catalytic efficiency. The information gained through XRD informs the design of new chemical processes and materials, paving the way for innovations in sustainable chemistry.
Mineralogy and Geology Applications
XRD is extensively used in mineralogy and geology for mineral identification and quantification. Geologists often face challenges when characterizing mineral phases in rock samples. XRD provides a non-destructive way to analyze such samples. This capability is critical in resource exploration, allowing for the assessment of ore deposits and understanding the geological history of regions.
Moreover, the technique is valuable in soil science. By understanding the mineral composition of soils, scientists can make informed decisions regarding land use and agriculture. It aids in determining nutrient availability and influences soil management practices, ultimately impacting crop yield and sustainability.
Biological and Pharmaceutical Uses
In the field of biology and pharmaceuticals, the applications of XRD are increasingly significant. XRD is utilized to characterize biomaterials and analyze drug formulations. For instance, in the pharmaceutical industry, understanding the crystallographic properties of active pharmaceutical ingredients can influence solubility and bioavailability.
Researchers apply XRD in the study of biomolecular structures as well. Analyzing protein crystallization provides insights into biological processes and aids in drug design. The ability to visualize molecular arrangements facilitates the development of targeted therapies and the understanding of disease mechanisms.
"XRD reveals structural details that are fundamental in guiding the rational design of new drugs."
In summary, XRD testing is integral across multiple fields, from materials science to pharmaceuticals. Understanding its applications enables stakeholders to harness its potential for advancing research and development.
Interpreting XRD Patterns
Interpreting XRD patterns is essential to understanding the structural properties of crystalline materials. This process involves analyzing the diffraction data produced by an X-Ray Diffraction (XRD) test. The resulting patterns reveal valuable insights into the material's composition and structure, which can be pivotal in a variety of scientific and industrial applications. Accurate interpretation allows researchers to deduce important characteristics like lattice parameters, crystallite size, and even the presence of impurities. This information is critical as it supports both fundamental research and practical applications in materials science, chemistry, and other related fields.
Understanding the XRD Spectrum
The XRD spectrum presents itself as a series of peaks, each corresponding to specific crystallographic planes of the material under investigation. The position and intensity of these peaks contain crucial information. The angle at which these peaks occur can be directly linked to the lattice structure of the substance, described by Bragg's Law. The intensity of the peaks relates to the density of the atoms in the lattice plane. Therefore, a clear grasp of how to read this spectrum is fundamental.
Key elements to consider in the XRD spectrum include:
- Peak Position: The specific angles where peaks appear help identify the phase of the material.
- Peak Intensity: The height of the peaks indicates how many atoms align in a particular direction, providing data on texture and preferred orientation.
- Peak Width: The breadth of the peaks can offer insight into crystallite size; narrower peaks often suggest larger crystals and vice versa.
Moreover, various software and databases can assist in translating the XRD patterns into comprehensible structural data. Familiarity with tools such as the ICDD database enables practitioners to match observed patterns with documented standards for accurate phase identification.
Phase Identification Techniques
Phase identification is a central aspect of interpreting XRD patterns. It involves differentiating between various crystalline phases present in the sample. Several techniques and methods are widely utilized for effective phase identification:
- Comparison to Standards: Matching the obtained patterns to the standard reference patterns available in databases is a common approach. The International Centre for Diffraction Data (ICDD) maintains a comprehensive collection of such standards.
- Indexing Peaks: This method involves assigning Miller indices to the observed peaks, based on their positions. Accurate indexing allows for the determination of the unit cell parameters of the crystal structure.
- Rietveld Refinement: This sophisticated technique refines the entire XRD pattern rather than just focusing on individual peaks. It allows for a precise extraction of structural information, including atomic positions and thermal vibrations.
- Machine Learning Models: With advancements in technology, machine learning approaches are increasingly utilized for phase identification. These models can rapidly compare numerous patterns and learn from data, improving accuracy and speed.
Focus on the phase identification aspect in XRD testing, combined with clear interpretation of the spectrum, enhances the overall understanding of material properties. This knowledge is not only essential for academic research but also carries significant implications for industries producing or utilizing crystalline materials.
Limitations of XRD Testing
While X-Ray Diffraction (XRD) offers valuable insights into material structures, it is not without limitations. Understanding these constraints is vital for both accurate interpretation of results and design of experiments. In this section, we highlight two significant aspects that affect the efficacy of XRD testing: challenges in sample analysis and limitations in data interpretation.
Challenges in Sample Analysis
The first area of concern lies in the complexities related to sample preparation and analysis. XRD requires samples to be homogeneous and finely powdered, which can pose problems for certain materials. Specimens that are too large or too coarse may lead to inaccurate results due to poor interaction with the X-ray beam. Furthermore, any impurities or non-crystalline phases in the samples can obscure the diffraction patterns, leading to misinterpretations.
Additionally, the technique is sensitive to sample orientation. If the crystalline grains are not uniformly oriented, the diffraction peaks might be weak or even absent, diminishing the reliability of the data collected. Furthermore, specific materials, such as those with preferred orientation, can result in anisotropic diffraction, which complicates the analysis.
Limitations in Data Interpretation
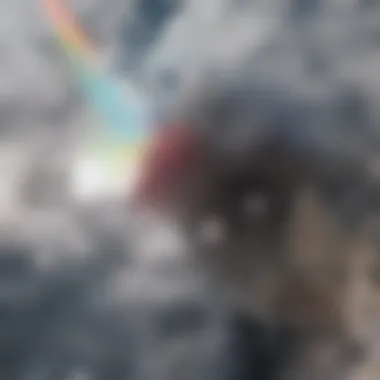
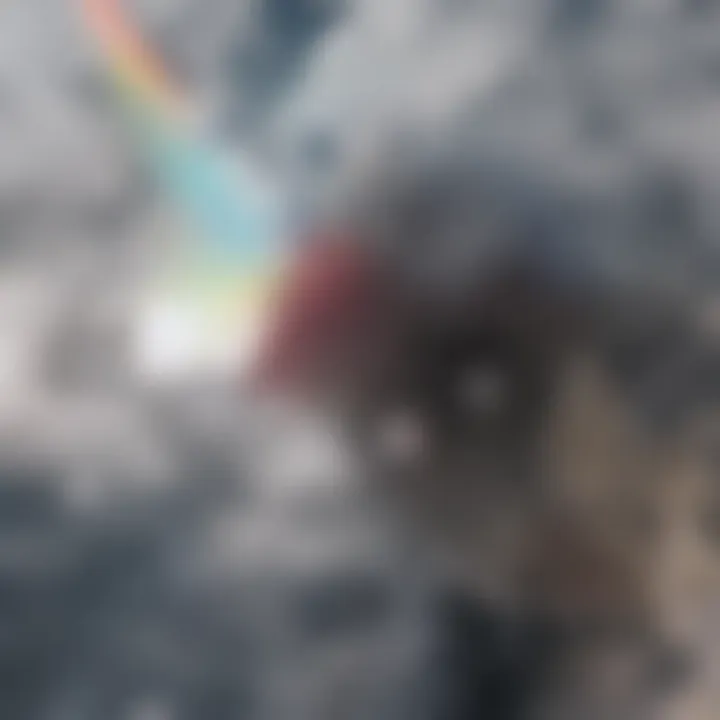
Interpreting XRD data also introduces its own set of challenges. XRD primarily provides information about the crystalline phases present in a sample, but it does not offer insights into the chemical composition. Thus, multiple phases can produce overlapping peaks, making it hard to distinguish between them without additional analytical techniques. This issue is particularly pronounced with complex multi-phase mixtures where the contribution of each phase needs further elucidation.
Moreover, the intensity of the diffracted peaks is affected by factors like crystallite size and strain, which can mask or distort the true representation of the sample’s structure. This leads to the potential for erroneous conclusions based solely on XRD results. Furthermore, XRD cannot provide information about the properties linked to non-crystalline materials, which can significantly limit its applicability in certain fields.
Ultimately, while XRD is a powerful tool for characterizing materials, it is crucial to recognize these limitations. A thorough understanding of the challenges in sample analysis and data interpretation can lead to more accurate assessments and inform the application of complementary analytical techniques as required.
"An awareness of the limitations inherent in X-ray diffraction testing is essential for researchers aiming to achieve high accuracy and validity in their findings."
.
Recent Advancements in XRD Technology
Recent advancements in X-Ray Diffraction (XRD) technology have significantly enhanced its application across various scientific fields. These advancements not only refine the accuracy of the testing but also broaden its utility in research and industry. With an emphasis on precision, speed, and data analysis, new technologies are poised to change how XRD is implemented.
Emerging Techniques in XRD
Emerging techniques in XRD include improvements in instrument design and data acquisition. One notable advancement is the development of high-throughput XRD systems. These systems allow for the rapid analysis of multiple samples simultaneously. This is beneficial in areas like materials science, where understanding the properties of various compounds quickly can lead to faster innovation.
Another noteworthy technique is the in-situ XRD, which allows scientists to observe the changes in a sample while it undergoes physical or chemical processes. This real-time analysis is crucial in understanding reaction pathways and material behavior under different conditions. It enables researchers to collect data that was previously difficult to obtain, enhancing the depth of information available from XRD studies.
Additionally, the integration of machine learning algorithms in data analysis represents a pivotal development. These algorithms can process large datasets efficiently, identifying patterns that might be missed by traditional methods. As a result, researchers can derive insights more quickly, even from complex spectra.
Integration with Other Analytical Methods
The integration of XRD with other analytical methods also marks a significant advancement. Techniques such as scanning electron microscopy (SEM) and transmission electron microscopy (TEM) are now often used in tandem with XRD. This combination provides complementary information—where XRD identifies phase information, SEM or TEM can provide detailed morphological data.
Furthermore, advancements such as XRD combined with spectroscopy techniques enhance the depth of analysis. For instance, coupling XRD with Raman spectroscopy can help in not only determining structural information but also gaining insights into vibrational modes of materials. This synergistic approach enables a multifaceted analysis of materials, thus broadening the scope of XRD applications.
In summary, the recent advancements in XRD technology, through emerging techniques and integration with other analytical methods, have reinforced its relevance and utility in modern scientific research. These innovations facilitate a deeper understanding of materials, contributing significantly to numerous fields.
Future Directions in XRD Research
The field of X-Ray Diffraction (XRD) research is evolving rapidly, as new technologies and methodologies emerge to enhance the capabilities of XRD testing. This section will examine the importance of future directions in this area, focusing on specific elements that will benefit both research and practical applications.
Research in XRD is not static. It has potential for significant innovations that will improve our ability to analyze materials on a microstructural level. Continued advancements in detection technology, software algorithms, and data analysis methods will play a crucial role in driving research forward. Future studies may include the integration of machine learning techniques, which can help identify patterns in complex datasets and improve the accuracy of phase identification.
Innovations in XRD Applications
Innovations in XRD applications hold great promise for several fields. As researchers continue to develop new techniques, they enhance the versatility of XRD. Some notable innovations are:
- In Situ Analysis: Real-time monitoring of phase changes during reactions can be achieved through advancements in XRD instrumentation.
- 3D X-Ray Diffraction: The development of techniques allowing for three-dimensional mappings can yield finer details about crystal orientations.
- Portable XRD Systems: Innovations are making XRD more accessible in field applications, such as geology and forensic science.
These innovations can transform industries by providing better analytical capabilities and faster results.
Potential Areas for Further Study
Several potential areas beckon further exploration within the realm of XRD research. Areas to consider include:
- Nanomaterials Characterization: Understanding the structures and behaviors of nanomaterials through XRD can contribute greatly to materials science.
- Hybrid Materials: Investigating composite materials where different phases coexist can unveil crucial performance characteristics.
- Environmentally Friendly Techniques: Developing eco-friendly methods for XRD testing could minimize waste and resources.
"The future of XRD lies in addressing complex materials systems and integrating advanced technologies that can enhance our interpretative capabilities."
Overall, future directions in XRD research could reshape how we analyze and understand material properties. Emphasis on innovation and addressing current limitations will lead to significant progress across multiple scientific domains.
Finale
The conclusion of this article encapsulates the significance of X-Ray Diffraction (XRD) testing. It serves as a synthesis of key elements discussed in previous sections, emphasizing the vital role of XRD in various scientific fields such as materials science, chemistry, and geology. One primary benefit of XRD is its ability to determine crystal structures, which is crucial for understanding material properties and behavior.
In this article, we reviewed foundational principles like Bragg's Law, methodologies for sample preparation, and applications in numerous domains. Each aspect underscores how integral XRD has become in modern research and industrial practices. The limitations of XRD were also addressed, providing a balanced view of the technology's strengths and challenges. Recognizing these limitations allows researchers to innovate and improve upon traditional methods.
Additionally, the discussion on recent advancements highlights how technological progress is pushing the boundaries of what XRD can achieve. As methods evolve, the relevance of XRD testing increases, offering new insights and applications.
Summarizing Key Takeaways
- XRD is essential for materials characterization, providing insights into structure and composition.
- The method is widely applicable across disciplines, which illustrates its versatility.
- Recent advancements enhance its capabilities, integrating with other analytical techniques.
- Awareness of limitations is crucial for proper application and interpretation of results.
This article aims to elevate the understanding of XRD, equipping readers to apply this knowledge effectively in their own research and professional activities.
The Ongoing Relevance of XRD Testing
XRD testing continues to hold relevance today due to its adaptability and precision. In an era where material innovation is paramount, XRD plays a fundamental role in material development and characterization. Researchers depend on this technique to discern complex structures in nanomaterials, polymers, and biomaterials, contributing to progress in various sectors, including pharmaceuticals and renewable energy.
Furthermore, the integration of XRD with other techniques like electron microscopy has broadened its scope. This amalgamation allows for comprehensive material analysis, facilitating deeper insights into material properties and behaviors.
As new challenges arise in materials science, XRD will likely evolve to meet these needs. The ongoing relevance of XRD testing lies in its ability to adapt and provide a foundational understanding necessary for future discoveries.
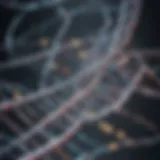
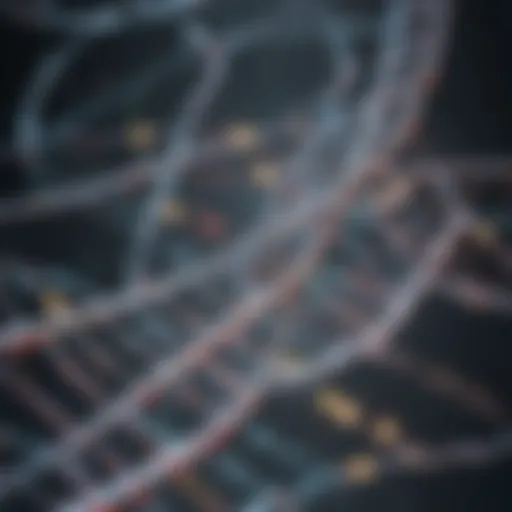