Understanding the Intricacies of Protein Synthesis
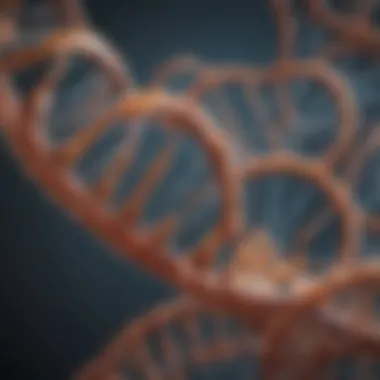
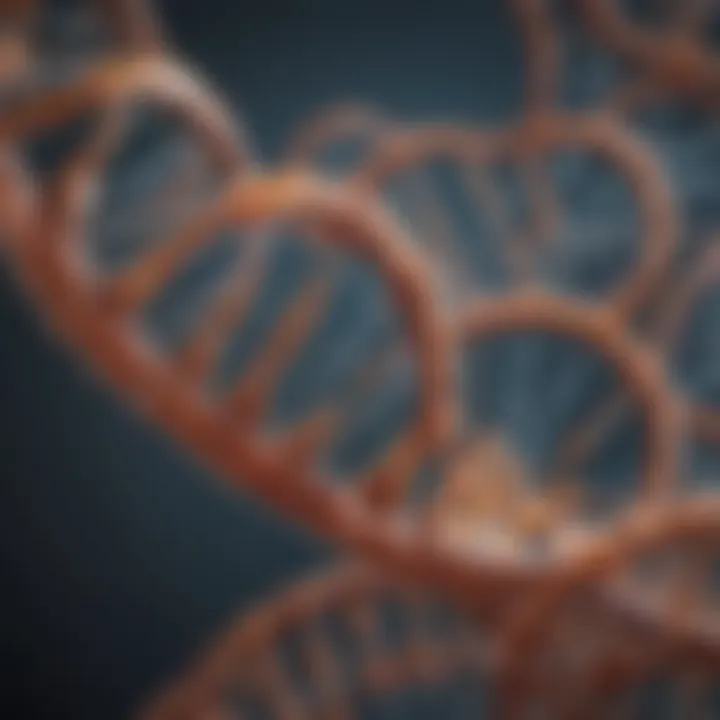
Intro
Understanding protein synthesis is foundational in molecular biology. This complex process translates genetic information into functional proteins, crucial for maintaining cellular integrity and function. Protein synthesis occurs in two main stages: transcription and translation, followed by several post-translational modifications. These processes are not isolated; they interact with various cellular components.
By examining each step in detail, researchers and students can appreciate the intricacies of how proteins are formed from DNA sequences. As we explore this subject, we will highlight significant methodologies in studying protein synthesis, along with the current technological advancements that enhance our comprehension of these cellular mechanisms. This will serve as both an informative guide and a resource for those seeking to deepen their understanding of molecular biology.
Preamble to Protein Synthesis
Protein synthesis is a fundamental biological process that lies at the heart of cellular function and life itself. Understanding this process is essential, as it involves the conversion of genetic information into functional proteins, which perform a vast array of tasks within organisms. Key elements of protein synthesis include transcription, translation, and post-translational modifications. This article seeks to elucidate these mechanisms, offering insights that benefit students, researchers, educators, and professionals alike.
Definition and Importance
Protein synthesis can be defined as the multi-step process through which cells generate proteins based on the instructions encoded in DNA. The importance of this process cannot be overstated. Proteins are critical for the structure and function of cells, acting as enzymes, structural components, and signaling molecules. This diversity of roles underscores the necessity of accurate and efficient protein synthesis, as any errors may lead to diseases or dysfunctions within the organism. Moreover, understanding protein synthesis enables advancements in fields such as genetics, biotechnology, and medicine.
Overview of Genetic Information
Genetic information is stored in the form of DNA, a molecule that contains the instructions for building proteins. The flow of genetic information within a cell follows a specific pathway often referred to as the central dogma of molecular biology: DNA is transcribed into messenger RNA (mRNA), which is subsequently translated into amino acid chains, ultimately folding into functional proteins. This process illustrates how information is processed and utilized within the cell.
Key Points:
- DNA carries the genetic blueprint.
- mRNA serves as the intermediary between DNA and proteins.
- The correct functioning of this pathway is vital for cellular health.
The Role of DNA
The role of DNA in protein synthesis is fundamental. It serves as the blueprint for the entire process, containing the genetic instructions necessary for producing proteins. Without DNA, the synthesis of proteins would not occur, as it provides the specific sequences of nucleotides that encode for amino acids, the building blocks of proteins.
DNA is organized into genes, each of which corresponds to a specific protein or function. These genes are not only essential for normal cellular operations but also for responding to environmental changes. This genetic blueprint enables cells to produce various proteins that perform critical roles, such as catalyzing metabolic reactions, facilitating cellular communication, and providing structural support.
Structure of DNA
The structure of DNA is a double helix, consisting of two strands twisted around each other. This structure is composed of nucleotides, each containing a phosphate group, a sugar molecule, and one of four nitrogenous bases: adenine, thymine, cytosine, or guanine. The specific pairing of these bases (adenine with thymine and cytosine with guanine) forms the rung-like structures in the double helix.
This double helical arrangement is crucial for DNA's stability and function. The sugars and phosphates form the backbone of the DNA strands, while the nitrogenous bases face inward. This orientation allows for the encoding of genetic information by forming complementary base pairs, which are essential during replication and transcription processes.
Gene Expression Regulation
Gene expression regulation is a complex set of mechanisms that determines when and how much of a particular protein is produced in a cell. This regulation is vital for maintaining cellular functions and responding to internal and external stimuli. Various factors influence gene expression, such as transcription factors, enhancers, and repressors, which can activate or inhibit the transcription of specific genes.
Additionally, epigenetic factors also play a significant role. Techniques like DNA methylation and histone modification can alter gene accessibility without changing the DNA sequence itself. This means that the same DNA can produce different amounts of proteins at different times or in different types of cells. Thus, gene expression regulation is paramount for cellular differentiation, development, and adaptation.
Understanding the role of DNA and its regulation is crucial to grasp how proteins are synthesized and how this process affects overall cellular function.
In summary, DNA's structural and regulatory functions are central to protein synthesis. By facilitating the proper expression of genes, DNA ensures that cells can produce the proteins necessary for life.
Transcription Process
Transcription is a crucial phase in the journey from DNA to protein. It serves as the first step in gene expression, converting DNA sequences into RNA. This step is essential, as it sets the stage for all subsequent processes in protein synthesis, including translation. Without a properly functioning transcription process, an organism would fail to produce the proteins necessary for life. Thus, understanding transcription provides insights into cellular functions, genetic regulation, and evolutionary biology.
Initiation of Transcription
The initiation of transcription begins when RNA polymerase binds to a specific region of the DNA known as the promoter. This region is usually located upstream of the gene. The binding of RNA polymerase is facilitated by various transcription factors which are proteins that help regulate the transcription process. Once RNA polymerase attaches to the promoter, it unwinds the DNA strands, creating a transcription bubble. The enzyme then begins to synthesize a complementary RNA strand by adding ribonucleotides that are complementary to the DNA template strand.
This step is tightly regulated. Only specific genes are transcribed at any given time, depending on signals from the environment or other cellular cues. The precise control of transcription initiation is vital for cellular differentiation and response to external stimuli.
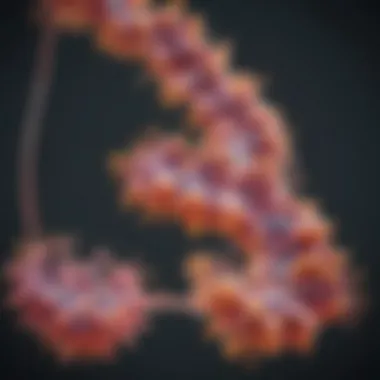
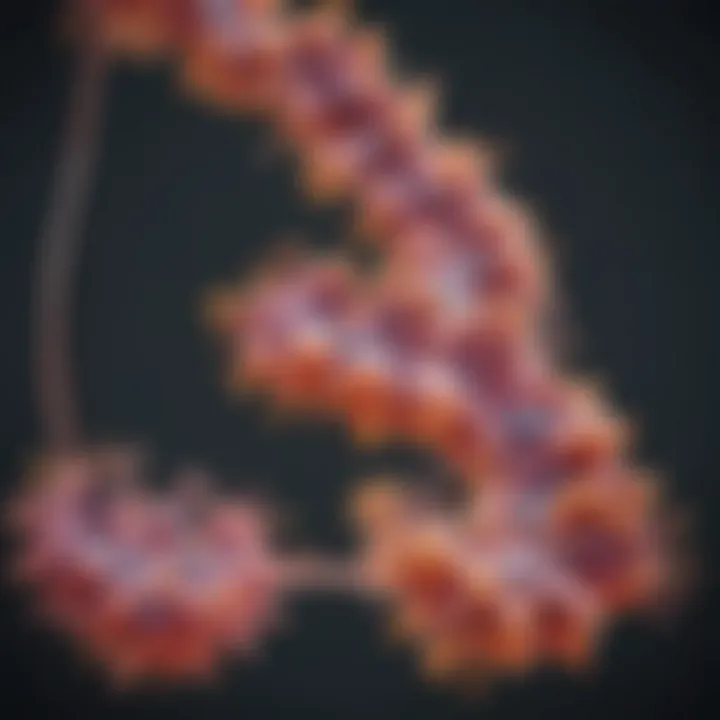
Elongation of RNA Strand
After initiation, the elongation phase starts as RNA polymerase moves along the DNA template strand. During elongation, the enzyme catalyzes the addition of ribonucleotides to the growing RNA chain. Each ribonucleotide pairs with its complementary DNA base – adenine pairs with uracil, while cytosine pairs with guanine. As RNA polymerase travels along the DNA, it continues to unwind the double helix, allowing access to further portions of the gene.
The elongation process is characterized by a rapid increase in RNA molecule length. The transcription rate can vary, influenced by the availability of nucleotides and the binding of various regulatory molecules. Additionally, the RNA strand being formed is a precursor to messenger RNA (mRNA), which carries information needed for protein synthesis.
Termination of Transcription
The termination of transcription involves several mechanisms that signal to RNA polymerase to stop synthesizing RNA. Specific sequences within the gene or external signals dictate the end of the transcription process. In bacterial cells, a termination signal often forms a hairpin loop structure in the RNA, causing RNA polymerase to disengage from the DNA template.
In eukaryotic cells, the process is generally more complex. Termination may involve cleavage of the newly formed RNA transcript by proteins. This cleavage is often followed by the addition of a polyadenylation signal, which helps in determining the stability and translation of the RNA.
Once transcription is complete, the RNA transcript undergoes further processing before it can function as mRNA, ready for the transition to the next stage of synthesis.
Proper regulation of transcription is essential for maintaining cellular functions and adapting to environmental changes.
RNA Processing
RNA processing is a critical phase in the journey from DNA to functional proteins. This process ensures that the RNA molecules produced from transcription are mature and functional before they are translated into proteins. Without proper RNA processing, the efficiency and accuracy of protein synthesis could be compromised. Understanding these mechanisms is essential for students and researchers who wish to grasp the complexities of molecular biology.
One of the main components of RNA processing is the modification of the RNA transcript to remove non-coding sequences, known as introns. The remaining sequences, called exons, are important for coding proteins. This selective splicing is crucial to producing the correct mRNA that will guide protein synthesis. In addition, RNA processing includes the addition of specific features that influence the stability and translational efficiency of the mRNA. Two significant modifications are the addition of the 5' cap and the poly-A tail, which enhance the integrity and functionality of the RNA molecule.
Splicing of Introns and Exons
Splicing is a vital step in RNA processing. It takes place in the nucleus and involves the removal of introns while joining together exons. Introns are non-coding regions that do not contribute to the final protein product. The ability to accurately excise these introns is essential for producing functional mRNA.
The splicing process is facilitated by a large complex called the spliceosome. This complex comprises small nuclear RNA (snRNA) and several protein components. The spliceosome recognizes specific sequences at the intron-exon boundaries, allowing it to precisely cut and rejoin RNA segments. The efficiency of this process can directly affect protein expression levels, as improperly spliced RNA may lead to the creation of nonfunctional proteins. Moreover, alternative splicing can produce multiple mRNA variants from a single gene, giving rise to different proteins with potentially diverse functions in the cell.
In summary, splicing ensures that only the relevant coding sequences are kept in the final mRNA.
Addition of ' Cap and Poly-A Tail
After splicing, the next phase of RNA processing involves the addition of the 5' cap and the poly-A tail. The 5' cap is a modified guanine nucleotide that is attached to the beginning of the mRNA strand. This modification serves several crucial purposes:
- Protection: The 5' cap shields the RNA from degradation by exonucleases, enzymes that can break down RNA.
- Regulation: It plays a role in the regulation of gene expression.
- Translation initiation: It helps ribosomes recognize the mRNA for translation.
On the other hand, the poly-A tail is a long stretch of adenosine nucleotides added to the 3' end of the mRNA. This tail contributes to:
- Stability: Like the 5' cap, the poly-A tail prevents degradation and prolongs the lifespan of the mRNA in the cytoplasm.
- Export: It assists in the export of the mRNA from the nucleus to the cytoplasm.
- Translation efficiency: It enhances the translation of the mRNA into proteins by promoting ribosomal binding.
Translation Process
Translation is a pivotal aspect of protein synthesis. This phase follows transcription and serves as the mechanism whereby the information encoded in messenger RNA (mRNA) is translated into a polypeptide chain, which eventually folds into a functional protein. Understanding translation is essential, as it directly influences the synthesis of proteins necessary for countless cellular functions. Additionally, exploring this topic reveals the intricate relationship between nucleic acids and the proteins they encode, shedding light on the fundamental processes that sustain life.
Initiation of Translation
The initiation of translation occurs when a ribosome assembles around the mRNA strand. Specifically, the small ribosomal subunit binds to the mRNA at the start codon, usually AUG, which codes for the amino acid methionine. This process is guided by various factors, such as initiation factors, which help stabilize the interacting components.
It is crucial to note that the correct initiation is vital for ensuring the fidelity of protein synthesis. A mistake during this step can lead to a malfunctioning protein, disrupting cellular processes. The proper binding of initiator tRNA is also essential as it sets the reading frame for the ribosome.
Amino Acid Chain Elongation
Once initiation is complete, the elongation phase begins. During elongation, amino acids are sequentially added to the growing polypeptide chain. This process involves three main sites on the ribosome: the A site, P site, and E site. The A site holds the incoming aminoacyl-tRNA, while the P site holds the tRNA that carries the polypeptide. During this phase, peptide bonds form as the ribosome shifts along the mRNA and catalyzes the addition of each new amino acid, extending the polypeptide chain.
The correctness of codon-anticodon pairing is indispensable during this stage. The ribosome checks the tRNA's anticodon against the mRNA's codon before accepting it. Furthermore, elongation requires elongation factors that facilitate the movement of the ribosome and the binding of tRNAs. This careful orchestration ensures that proteins are synthesized accurately and efficiently.
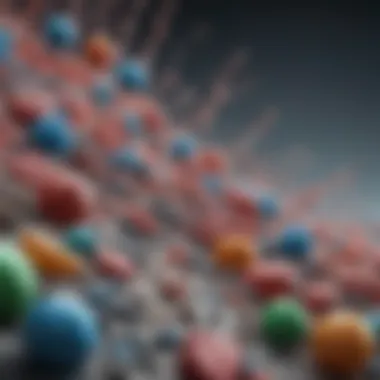
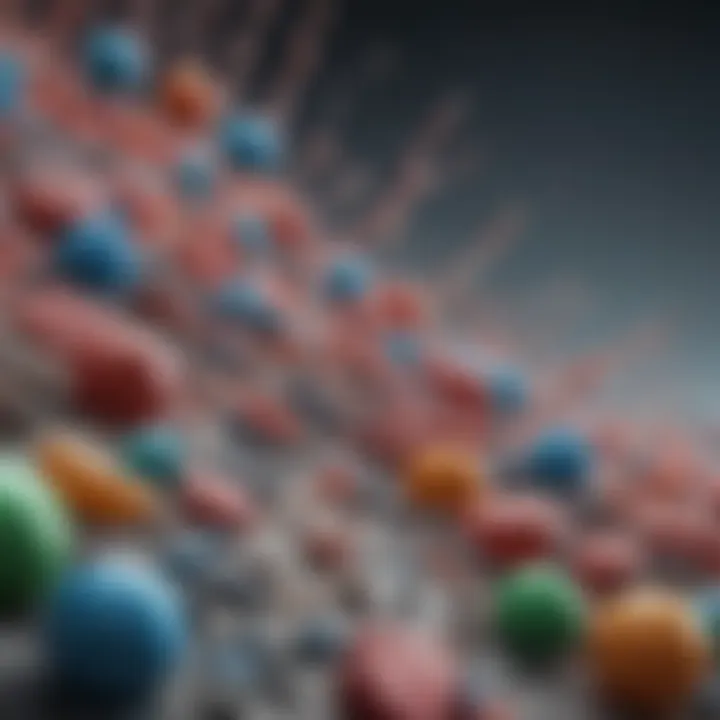
Termination of Translation
Termination occurs when the ribosome encounters a stop codon on the mRNA, which signals the end of the translation process. Stop codons—such as UAA, UAG, or UGA—do not correspond to any amino acid. Instead, release factors enter the ribosome, prompting the disassembly of the ribosomal subunits and the release of the newly formed polypeptide.
The termination step is critical, as it concludes the synthesis of the protein and allows for its proper folding and post-translational modifications. Failure to terminate correctly can lead to incomplete or dysfunctional proteins.
The translation process embodies the final and perhaps most critical step in protein synthesis, culminating the journey from DNA to functional proteins crucial for every aspect of cellular life.
Understanding the translation process equips researchers and students with insights into protein synthesis intricacies and its overarching impact on cellular biology. It forms a knowledge base that highlights potential areas for further study in genetics, biotechnology, and medicine.
Role of Ribosomes
Ribosomes are essential cellular components that play a pivotal role in the process of protein synthesis. These molecular machines facilitate the translation of mRNA into polypeptide chains, which subsequently fold into functional proteins. Understanding ribosomes provides insights into their structure, function, and the intricate processes occurring during translation. Their relevance to cellular biology cannot be overstressed, as they are fundamental to the functioning of all living cells.
Structure of Ribosomes
Ribosomes are composed of ribosomal RNA and proteins, forming a complex that can be classified into two subunits: the small subunit and the large subunit. In eukaryotic cells, the small subunit is known as 40S, while the large subunit is 60S, resulting in an overall 80S ribosome. In contrast, prokaryotic cells contain 30S and 50S subunits, which assemble into a 70S ribosome. This difference in structure is crucial for the functioning of specific ribosomal activities.
Each ribosomal subunit contains distinct sites for tRNA binding and polypeptide synthesis.
- The A (aminoacyl) site is where incoming tRNA delivers amino acids.
- The P (peptidyl) site holds the tRNA carrying the growing polypeptide chain.
- The E (exit) site is where empty tRNA exits the ribosome after its amino acid has been added to the chain.
The arrangement of these sites facilitates the assembly of amino acids into a polypeptide, making ribosomes essential for protein synthesis. The structural integrity of ribosomes is vital to maintain their function and efficiency.
Ribosomal RNA Function
Ribosomal RNA (rRNA) forms the core of the ribosome's structure and is crucial for its function. It provides a scaffold for the assembly of ribosomal proteins and is responsible for the catalytic activity of the ribosome. Unlike mRNA and tRNA, rRNA does not serve as a template but instead contributes to the overall architecture and functional capability of the ribosome.
The primary functions of rRNA include:
- Catalytic activity: rRNA facilitates the formation of peptide bonds between amino acids, which links them together in a polypeptide chain.
- Decoding function: rRNA plays a role in recognizing the codons on the mRNA, ensuring the correct tRNA is selected to match the encoded amino acid.
- Structural stability: The rRNA's structure enables ribosomes to withstand various stressors in the cellular environment, maintaining their functionality.
Transfer RNA (tRNA)
Transfer RNA, commonly known as tRNA, plays a pivotal role in the process of protein synthesis. It serves as a critical adaptor molecule that translates the genetic information encoded in messenger RNA (mRNA) into actual proteins. Without tRNA, the translation of nucleotide sequences into amino acid chains would not be possible, highlighting its essential function in cellular biology.
tRNA Structure and Function
The structure of tRNA is highly specialized. It has a characteristic cloverleaf shape, created by a series of folds and base pairings within a single RNA strand. This unique form enables tRNA to effectively interact with both mRNA and ribosomes during protein synthesis.
At one end of the tRNA molecule is the anticodon region. This region consists of three nucleotides that base-pair with the complementary codon on the mRNA. At the opposite end, tRNA carries a specific amino acid corresponding to its anticodon. This group of structures ensures that the correct amino acids are brought in sequence as specified by the mRNA.
The specific pairing of tRNA anticodons with mRNA codons is crucial for the accurateness of protein synthesis. Errors in this pairing can lead to the production of faulty proteins, which can affect cell function and overall organism health.
Aminoacyl-tRNA Synthetases
Aminoacyl-tRNA synthetases are vital enzymes that link amino acids to their appropriate tRNA molecules in a process known as aminoacylation. Each amino acid has a corresponding enzyme responsible for this attachment. This process occurs prior to translation and is crucial to ensure proper protein synthesis.
The mechanism involves two main steps:
- Activation of the amino acid: The enzyme catalyzes the reaction between an amino acid and ATP, resulting in the formation of an aminoacyl-AMP and the release of pyrophosphate.
- Attachment to tRNA: The amino acid is transferred from aminoacyl-AMP to the appropriate tRNA, creating an aminoacyl-tRNA complex.
This enzyme specificity is essential in maintaining fidelity during protein synthesis. If an incorrect amino acid is paired with a tRNA, it can drastically alter the structure and function of the resulting protein.
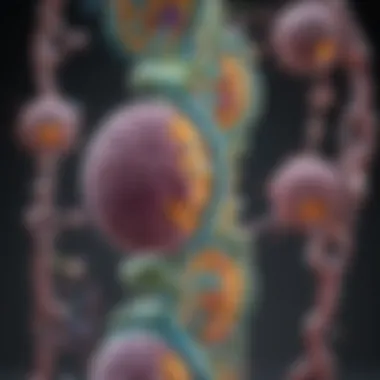
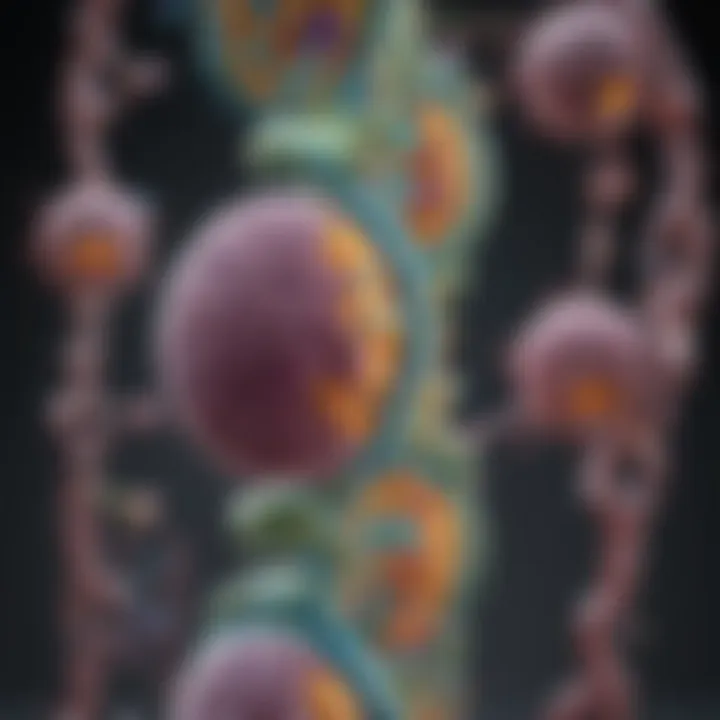
Overall, tRNA and aminoacyl-tRNA synthetases work in unison to facilitate the translation of genetic information into functional proteins, bridging the gap between nucleic acids and polypeptides. Their roles illustrate the complexity and precision involved in the cellular machinery that drives life.
Post-Translational Modifications
Post-translational modifications are critical for determining the functionality and lifespan of proteins within a cell. These processes occur after the initial synthesis of proteins and involve various chemical changes that alter a protein's structure or activity. Understanding these modifications is essential, as they can significantly impact cellular function and response to environmental signals.
Types of Modifications
There are several notable types of post-translational modifications that proteins may undergo:
- Phosphorylation: The addition of a phosphate group, frequently regulating protein activity and signaling pathways. This modification can either activate or deactivate enzyme activity and is integral in processes like cell division.
- Glycosylation: The attachment of carbohydrate groups, which aids in protein folding and stability. Glycosylated proteins often play critical roles in cell recognition and signaling pathways.
- Ubiquitination: The attachment of ubiquitin molecules, marking proteins for degradation. This process is vital for regulating protein levels and removing damaged or misfolded proteins from the cell.
- Methylation: The addition of methyl groups that often influences gene expression and protein interactions. This modification can change the activity of a protein, affecting its role in cellular processes.
- Acetylation: The addition of an acetyl group, typically impacting protein stability and interactions. Acetylation is known for its role in regulating gene expression by modifying histones in chromatin.
Each of these modifications carries distinct biochemical implications and reflects the dynamic nature of cellular regulation.
Role in Protein Functionality
Post-translational modifications serve to expand the functional repertoire of proteins beyond what is encoded in the genome. They provide an additional layer of regulation that is essential for cellular adaptation and response. The implications of these modifications include:
"Post-translational modifications determine the functional states of proteins, affecting everything from enzyme activity to signal transduction."
- Activity Regulation: Many proteins only become active after specific modifications, influencing pathways such as metabolism and signal transduction.
- Localization Control: Modifications can alter a protein's location within a cell, which is critical for proteins that function in different cellular compartments.
- Stability and Turnover: Modifications like ubiquitination play a key role in determining how long a protein remains functional within the cell, hence influencing the overall protein turnover.
- Cell Communication: Glycosylated proteins are important in cell surface interactions, which are necessary for immune response and cell signaling.
Among these roles, it stands clear that post-translational modifications are not merely decorative but rather are instrumental in shaping the destiny and efficiency of proteins in biological systems. They contribute fundamentally to the intricate network of cellular functions and underscore the complexity of protein behavior.
Summary of Protein Synthesis
The summary of protein synthesis ties together the various components discussed in this article. Understanding this process is crucial for various fields like molecular biology, genetics, and biochemistry. Protein synthesis is not merely a sequence of events; it is a fundamental mechanism by which living cells create proteins that determine their structure and function. This narrative elucidates the steps of protein synthesis, each playing a pivotal role in cells' viability and efficacy.
Recap of Key Steps
Protein synthesis involves several well-defined stages: transcription, translation, and post-translational modifications. These phases can be briefly summarized as follows:
- Transcription: This is the initial stage where the DNA sequence of a gene is transcribed to create messenger RNA (mRNA). This mRNA carries the genetic instructions from the DNA in the nucleus to the ribosomes in the cytoplasm.
- Translation: During this phase, the ribosomes decode the mRNA sequence to synthesize a specific polypeptide chain. Transfer RNA (tRNA) plays a critical role here, bringing the appropriate amino acids to the ribosome, facilitating the assembly of the protein.
- Post-Translational Modifications: Once synthesized, proteins can undergo various modifications that affect their activity, stability, and localization within the cell. These processes include glycosylation, phosphorylation, and other chemical changes that enhance protein functionality.
By recognizing these steps, researchers can gain insights into how proteins are formed and how alterations in this process can lead to various diseases or conditions.
Implications for Cell Biology
The implications of protein synthesis span far beyond simple molecular mechanics. They touch on critical aspects of cellular biology, such as:
- Cell Growth and Repair: Proteins are essential for cellular structure and function. They support growth, repair, and the maintenance of cellular functions, making protein synthesis a vital process for cellular integrity.
- Gene Expression: The regulation of protein synthesis is essential for gene expression. Any disturbance in this regulation can lead to aberrant protein production, impacting cellular functions and leading to diseases.
- Biotechnological Applications: Understanding protein synthesis has profound implications in biotechnology. Proteins are used in pharmaceuticals, diagnostics, and food technology. Knowledge of how to manipulate this process can enhance productivity in these sectors.
"Protein synthesis is fundamental to life's processes, shaping organismal structure and function."
Ending
In concluding this article, it is essential to comprehend the totality of protein synthesis and its quantifiable importance in biological systems. Protein synthesis exhibits a precise orchestration of events from transcription to translation and ultimately to post-translational modifications, all of which culminate in the formation of functional proteins. Understanding these processes is instrumental for multiple fields, including molecular biology, genetics, and biotechnology.
Future Directions in Protein Research
Research into protein synthesis is continually evolving. Future directions may include advancements in synthetic biology, where scientists aim to design and construct new biological parts, devices, and systems. This could lead to more effective therapies for genetic diseases by directly modifying the protein synthesis pathways.
Additionally, the study of riboswitches—segments of RNA that can regulate gene expression—offers potential. These findings could enhance our understanding of gene regulation, ultimately leading to breakthroughs in medicine and agriculture.
Moreover, computational tools are becoming more sophisticated, allowing researchers to predict protein structures and functions from amino acid sequences. This could radically transform how we approach drug design and development.
The Evolution of Our Understanding
Our understanding of protein synthesis has evolved significantly over the past century. Early scientists established the core concepts of DNA as the genetic material, but several breakthroughs have since refined our comprehension. The deciphering of the genetic code was a seminal moment, unlocking how sequences of nucleotides correspond to specific amino acids.
Furthermore, discoveries surrounding ribosomes, tRNA, and the role of various enzymes have painted a more complete picture. With ongoing research, we are gradually uncovering the complexities within the regulation of protein synthesis. This evolution broadens the horizon not only for fundamental science but also for its implications in health, disease, and biotechnology.