Understanding the Basics of Polymerase Chain Reaction
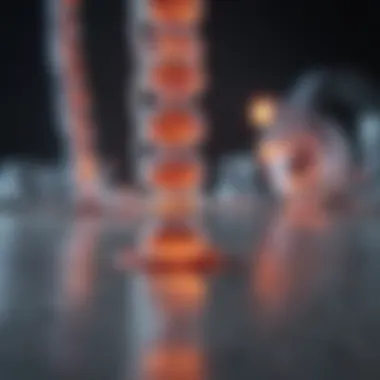
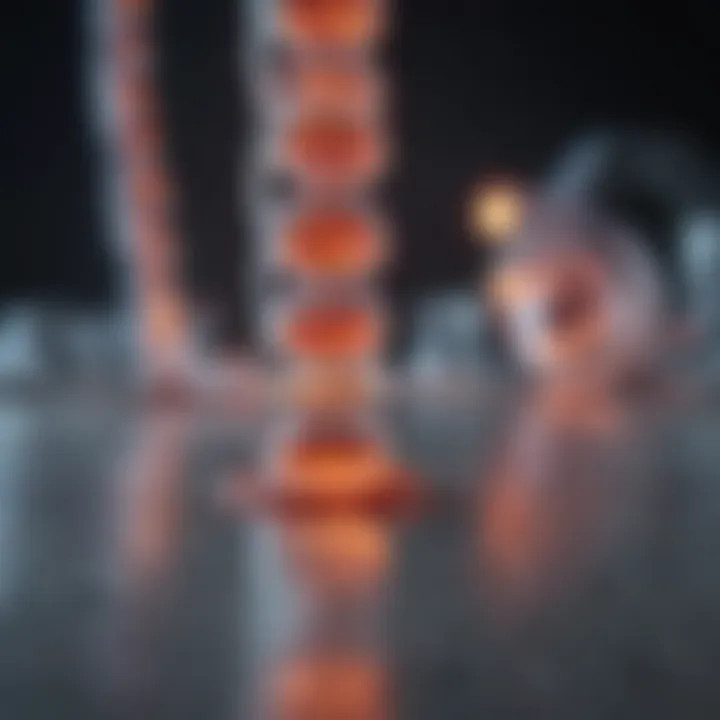
Intro
Polymerase Chain Reaction (PCR) has transformed molecular biology by allowing scientists to amplify specific DNA sequences. This process is fundamental in various applications, ranging from clinical diagnostics to genetic research. Understanding PCR's principles enhances comprehension of its impact and significance in modern science.
This article will provide a detailed examination of PCR methodologies, tools, and technologies. It will also discuss limitations and potential advancements while ensuring the content is suited for students, researchers, and professionals in the field.
Methodologies
Description of Research Techniques
PCR operates on simple yet effective principles. The basic cycle of PCR includes three main steps: denaturation, annealing, and extension. During denaturation, the double-stranded DNA melts open into two single strands when heated to around 94-98 °C. This step is crucial as it separates the DNA strands, making them available for the following processes.
Once denaturation is complete, the temperature cools to 50-65 °C, allowing primers to hybridize to the target DNA sequence during the annealing phase. Primers are short sequences of nucleotides that initiate DNA synthesis. An improper primer design can lead to low yield or non-specific amplification. In the final step, extension occurs as the temperature is raised to about 72 °C. During this phase, DNA polymerase synthesizes a new DNA strand by adding nucleotides to the primers, thereby amplifying the target DNA sequence exponentially.
Tools and Technologies Used
Several tools and technologies facilitate PCR execution. The key components include:
- Thermal Cyclers: Instruments that precisely control the temperature transitions required for the PCR process. They are essential for automating the heating and cooling cycles.
- DNA Polymerases: Enzymes such as Taq polymerase, derived from the thermophilic bacterium Thermus aquaticus, are responsible for synthesizing new DNA strands. Their high-temperature tolerance is vital for efficient PCR.
- Primers: Custom-designed short nucleotide sequences that bind specifically to the target DNA. Their specificity is critical for successful amplification.
- Buffers and Dyes: Buffers provide the necessary pH and ionic strength for the reaction, while dyes can be used to visualize the PCR products directly.
PCR has evolved, leading to various adaptations like real-time PCR, multiplex PCR, and quantitative PCR, which offer improved sensitivity and specificity.
Discussion
Comparison with Previous Research
Previously, DNA amplification was challenging and often required complex and time-consuming procedures. Early methods, like cloning, could only produce limited yields and were more labor-intensive. PCR’s introduction offered a rapid and efficient method for amplifying DNA, significantly advancing genetic research and diagnostics.
Theoretical Implications
PCR has substantial theoretical implications in genetics and molecular biology. It allows researchers to explore genetic variations, study gene expression, and even identify pathogens. As PCR technology improves, it opens doors for innovative research directives, promising to unveil more intricate biological mechanisms at play.
PCR remains a cornerstone technique in molecular biology, emphasizing the role of efficient DNA amplification in scientific discovery and diagnosis.
In summary, understanding PCR's methodologies not only enhances knowledge of molecular techniques but also underscores its importance in advancing biological sciences. This foundational knowledge is invaluable for anyone engaged in research, diagnostics, or education within the life sciences.
Prelims to PCR
Polymerase Chain Reaction (PCR) stands as a cornerstone in modern molecular biology, showcasing its importance in various scientific fields including genetics, microbiology, and forensics. Understanding PCR is essential not just for those directly involved in laboratory work, but also for professionals who rely on its applications in diagnostics and research. The capability of PCR to amplify specific DNA segments with high precision has transformed how scientists analyze genetic material. This introductory section lays the foundation for understanding the intricate mechanisms and breakthroughs that PCR has made possible.
Definition of PCR
Polymerase Chain Reaction (PCR) is a widely used technique that allows for the amplification of a specific segment of DNA. Introduced by Kary Mullis in the 1980s, PCR enables the generation of millions of copies of a particular DNA sequence in a matter of hours. The process relies on repeated cycles of heating and cooling, facilitating the denaturation of DNA, annealing of primers, and extension of new DNA strands through the action of DNA polymerases. This remarkable ability to multiply DNA makes PCR an invaluable tool in multiple areas such as genetic testing, cloning, and pathogen detection.
Historical Background
The history of PCR is fundamental to understanding its impact on science and medicine. The technique was first developed by Kary Mullis in 1983, who envisioned a method to replicate specific DNA strands without the need for bacterial culture. It was a revolutionary approach at the time, as previous methods for DNA amplification were labor-intensive and inefficient. Mullis’s invention laid the groundwork for rapid and reproducible DNA amplification.
In 1991, the first commercial PCR machine was introduced, significantly improving accessibility to this technology. As researchers began to utilize PCR for various applications, it quickly gained traction in clinical diagnostics and genetic research. The integration of PCR into clinical labs has since paved the way for precise disease diagnosis and personalized medicine.
Today, PCR continues to evolve, with advancements including Real-Time PCR and Digital PCR, expanding its applications and improving efficiency. The historical journey and continued development of PCR highlight its significance and the ongoing quest for innovation in molecular biology.
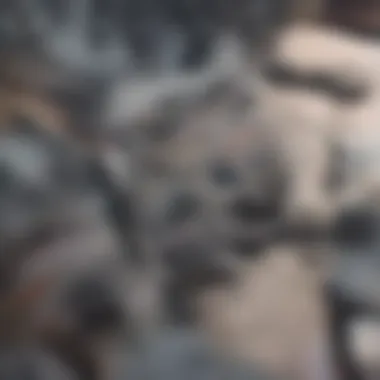
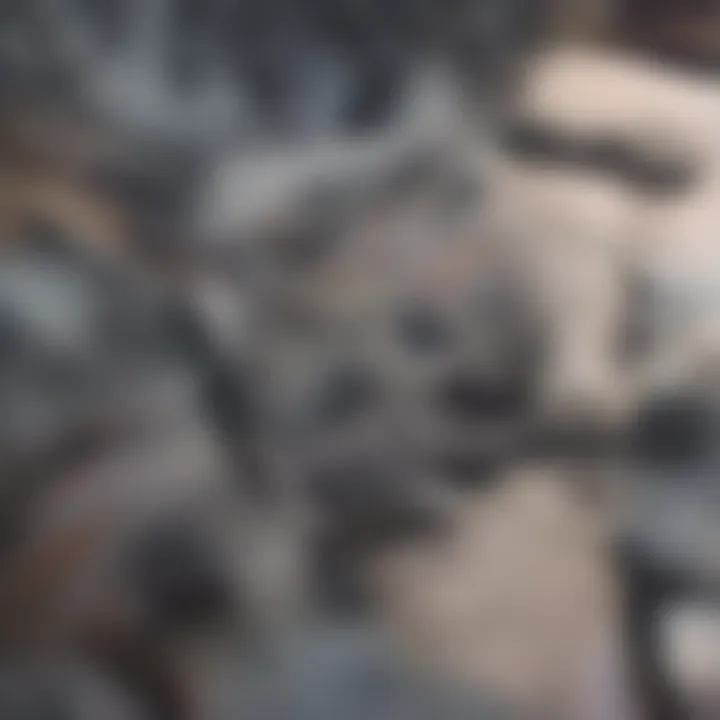
"PCR has fundamentally changed the way we approach molecular diagnostics and gene analyses, amplifying not just DNA but also our potential to understand life at the molecular level."
By familiarizing ourselves with PCR's definition and historical context, we equip ourselves to delve deeper into the fundamental principles that govern its operation.
Fundamental Principles of PCR
The fundamental principles of Polymerase Chain Reaction (PCR) are essential for understanding how this innovative technique functions. First developed by Kary Mullis in the 1980s, PCR has revolutionized molecular biology by enabling the amplification of specific DNA sequences. This section will provide insight into the key elements that underpin PCR, emphasizing its components and their significance.
DNA Structure and Function
DNA, or deoxyribonucleic acid, serves as the hereditary material in all known living organisms. Its structure is a double helix composed of two strands, each made up of nucleotides. Each nucleotide contains a phosphate group, a sugar molecule, and one of four nitrogenous bases: adenine, thymine, cytosine, or guanine. The sequence and arrangement of these bases encode genetic information, which is vital for cellular function and organismal development.
Within the context of PCR, it is particularly crucial to comprehend how DNA can be located and amplified. Primers are short sequences of nucleotides designed to flank the target region of the DNA that researchers wish to amplify. Understanding the structure of DNA allows for the thoughtful design of these primers, ensuring they bind specifically to the desired segment, thus enhancing the sensitivity and accuracy of the amplification process.
"PCR can generate millions of copies of a specific DNA segment from a small sample, making it an indispensable tool in biotechnology."
Enzymatic Components of PCR
The success of PCR relies heavily on a few key enzymatic components. The most critical of these is DNA polymerase, an enzyme that synthesizes new strands of DNA by adding nucleotides complementary to the existing template strand. Taq polymerase, derived from the thermophilic bacterium Thermus aquaticus, is the most commonly used enzyme in standard PCR procedures. Its ability to withstand the high temperatures required for denaturation makes it ideal for preparing DNA for amplification.
Besides DNA polymerase, two additional reagents are critical in the PCR process:
- Primers: As mentioned earlier, these short, single-stranded DNA sequences provide a starting point for the polymerase to initiate synthesis. The specificity of the primers is key in ensuring that only the desired region of DNA is amplified.
- Deoxynucleotide triphosphates (dNTPs): These are the building blocks of DNA. Each dNTP consists of a deoxyribose sugar, a phosphate group, and one of the four nitrogenous bases. The availability of sufficient dNTPs is necessary for the synthesis of the new DNA strands during PCR.
In summary, a solid understanding of DNA structure and the enzymatic components essential to PCR is invaluable. Researchers can optimize conditions, enhance reproducibility, and successfully execute amplification of DNA of interest by grasping these principles.
The PCR Process
The PCR process is integral to the understanding of polymerase chain reaction methodology. This section highlights not just the mechanics involved but also the broader implications of the process itself. Grasping how PCR functions is crucial for its applications in various fields like genetics, diagnostics, and forensics. Each stage contributes significantly to the overall purpose of amplifying DNA, which is the core objective of PCR.
Stages of PCR
Denaturation
Denaturation is the first stage in the PCR process. Here, the double-stranded DNA is heated to a high temperature, typically around 94 to 98°C. The primary objective of this step is to separate the two strands of DNA, allowing access to the nucleotide sequences for replication. The unique feature of denaturation lies in its speed and efficiency. This quick heating process is beneficial as it ensures that the strands are entirely separated, preventing re-annealing.
However, it is essential to maintain precise temperature control. If the temperature is too high or maintained for too long, it may denature the DNA polymerase enzyme, rendering the process ineffective. The balance of temperature and time makes denaturation a critical phase in the overall PCR procedure.
Annealing
Following denaturation is the annealing stage, where the temperature is lowered to around 50 to 65°C. During this time, short sequences of primers bind to the target DNA strands. This specific pairing is crucial as it lays the groundwork for DNA synthesis in the next step. The key characteristic of annealing is its ability to ensure specificity in PCR.
The temperature during annealing affects the binding efficiency of the primers. A lower temperature might allow non-specific binding, while a higher temperature may inhibit the binding altogether. Therefore, it is important to optimize this step to ensure that only complementary sequences are targeted, enhancing the specificity of the PCR process.
Extension
The last stage is extension, where the temperature is adjusted to approximately 72°C. At this stage, the DNA polymerase enzyme synthesizes new DNA strands by adding nucleotides to the primers. The unique aspect of extension is its speed; many polymerases can add nucleotides at a rapid rate, allowing for efficient replication of the target DNA.
This step's efficiency significantly contributes to the yield of the PCR process, resulting in a large number of copies from a small initial amount of DNA. However, the quality of the DNA polymerase used is crucial here. If the enzyme is of low quality, it might introduce errors, leading to mutations in the copied DNA. Hence, selecting a high-fidelity polymerase is essential.
Temperature Cycling
Temperature cycling is the heart of PCR, orchestrating the transitions between the stages. This cycling consists of alternating between high and low temperatures to facilitate denaturation, annealing, and extension. The precise control of these cycles is what enables PCR to exponentially amplify the target DNA.
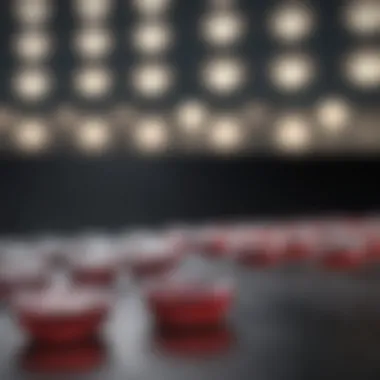
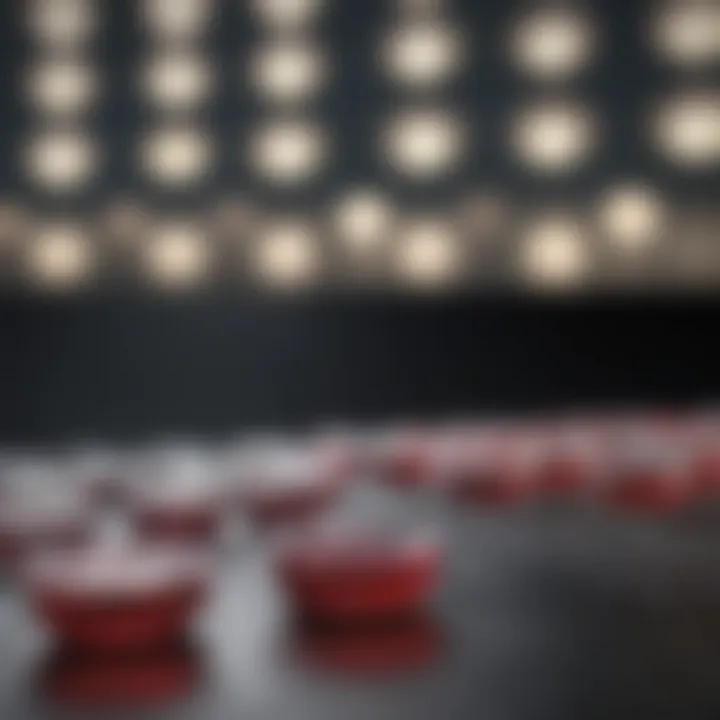
Each cycle typically takes several minutes, and the number of cycles can vary according to the specific requirements of the experiment or application, often ranging from 25 to 40 cycles. It is critical to recognize that while the process appears simple, the nuances of temperature cycling contribute immensely to the effectiveness of PCR. \n In summary, the PCR process, through its stages of denaturation, annealing, and extension, interlinks meticulously to create a reliable method for amplifying DNA. The understanding of this process not only aids in practical applications but also in comprehending advanced techniques in molecular biology.
Applications of PCR
Polymerase Chain Reaction (PCR) has revolutionized many areas of molecular biology, making it an essential tool for scientists around the world. Its versatility allows it to be applied in various fields, significantly impacting clinical diagnostics, genetic research, and forensic science. Understanding the applications of PCR illuminates its critical role in advancing scientific knowledge and improving practical outcomes.
Clinical Diagnostics
In the realm of clinical diagnostics, PCR plays a pivotal role in the identification of pathogens. This method has become fundamental for detecting diseases at an early stage. For instance, PCR is commonly used to diagnose infectious diseases like SARS-CoV-2, whereby a small amount of viral RNA can be amplified, enabling detection even in minuscule quantities.
Additionally, PCR aids in the identification of genetic disorders. By amplifying specific gene sequences, healthcare professionals can analyze mutations associated with various conditions. This genetic insight allows for better diagnosis and can inform treatment options, ensuring patients receive tailored care.
"PCR transforms how we approach the diagnosis of diseases, leading to quicker and more accurate results."
Genetic Research
In genetic research, PCR has become a indispensable method. It facilitates the amplification of DNA segments to study specific genes, aiding in the exploration of genetic functions and interactions. This process is essential for various research applications including gene cloning, sequencing, and genotyping. Researchers utilize PCR to investigate the role of specific genes in development, disease, and evolution.
Moreover, PCR is crucial for the study of genetic diversity within populations. By analyzing genetic variations, scientists can understand evolutionary relationships and species dynamics. PCR thus contributes significantly to fields like ecology and conservation biology, where understanding genetic variation is vital for species preservation.
Forensics
PCR's role in forensics is transformative, particularly regarding criminal investigations. It allows for the amplification of trace DNA found at crime scenes, which might otherwise be insufficient for analysis. The ability to analyze minute samples, such as hair strands or skin cells, enhances the chances of identifying suspects or victims.
DNA profiling using PCR has also streamlined the process of establishing identity in legal scenarios, proving vital in exonerating the falsely accused or confirming the identity of victims. Furthermore, advancements in PCR techniques, such as multiplex PCR, enable the simultaneous amplification of multiple DNA targets, improving efficiency in forensic analysis.
In summary, the applications of PCR extend far beyond laboratory settings. They encompass vital contributions to healthcare, research, and law enforcement. As technology progresses, the potential for PCR applications will likely continue to expand, offering innovative solutions to pressing challenges in society.
Variations and Advances in PCR
The field of molecular biology is dynamic and continuously evolving. Variations and advances in Polymerase Chain Reaction (PCR) technology represent a significant aspect of this evolution. Understanding these developments provides insight into how PCR adapts and improves, leading to enhanced accuracy and efficiency in various applications.
Real-Time PCR
Real-Time PCR, also known as quantitative PCR (qPCR), is a highly sensitive and efficient method of measuring DNA amplification as it occurs. This technique allows researchers to track the progress of the reaction in real time, which enhances the precision of quantifying DNA. Unlike traditional PCR, which measures the end product, Real-Time PCR provides data throughout the amplification process.
The use of fluorescent dyes or probes in Real-Time PCR is pivotal. As DNA amplifies, the fluorescent signal increases proportionally, offering a clear visual representation of the reaction. Key advantages include reduced risk of contamination and enhanced specificity. However, careful optimization is necessary for primer and probe selection as well as reaction conditions to avoid non-specific signals.
Inverse PCR
Inverse PCR is a technique designed to amplify DNA segments that are located adjacent to known sequences, thus allowing for the study of flanking regions. This technique is particularly useful when analyzing genomic DNA where direct amplification of targeted sequences is challenging.
In inverse PCR, a specific restriction enzyme digests the genomic DNA, followed by self-ligation of the fragments. Unique primers are then used to amplify the regions flanking the cut sites. The process is significant for applications in cloning, mapping genomic sequences, and studying genetic variations. A major consideration with inverse PCR is the need for meticulous primer design and optimization of reaction conditions to achieve successful amplification.
Digital PCR
Digital PCR represents a revolution in the quantitative capabilities of PCR by providing absolute quantification of nucleic acids without the need for standard curves. This technology involves partitioning a PCR reaction into thousands of individual reactions, allowing for more accurate quantification of low-abundance targets. Each partition either contains the target DNA and produces a detectable signal or does not. The results are then analyzed to calculate the number of target molecules present.
Digital PCR is notably useful in clinical diagnostics, rare mutation detection, and applications that require precise measurement of nucleic acids. One must consider that digital PCR can be more complex and costly than conventional methods, requiring advanced instruments and careful experiment design.
"The advances in PCR, particularly Real-Time, Inverse, and Digital PCR, demonstrate the potential and versatility of the technique, promoting innovation in both diagnostic and research applications."
In summary, these variations of PCR show the versatility and adaptability of PCR technology in a constantly evolving field. The implications of these advancements extend across basic research, diagnostics, and therapeutic monitoring, making them important tools in the toolkit of modern molecular biology.
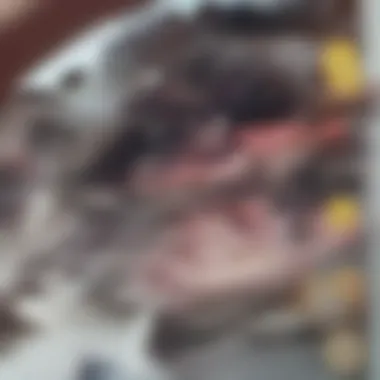
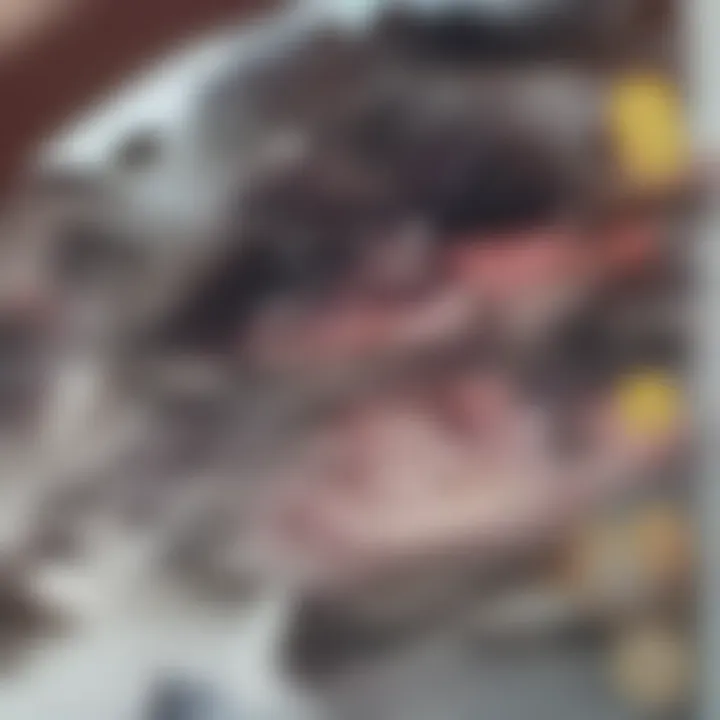
Limitations of PCR
Polymerase Chain Reaction (PCR) serves as a cornerstone technique in molecular biology, yet it is crucial to acknowledge its limitations. Understanding these shortcomings is essential for students, researchers, and professionals who rely on PCR for analyses and experiments. Awareness of such limitations allows for better experimental design and interpretation of results.
Specificity Issues
One significant limitation of PCR is its specificity. PCR amplifies DNA sequences based on the primers used. However, if the primers are not adequately designed, they may bind to non-target sequences, leading to nonspecific amplification. This phenomenon can obscure results and lead to inaccuracies in data interpretation. Factors that contribute to specificity issues include:
- Primer Design: Suboptimal primer sequences can lead to amplification of similar sequences rather than the intended target. This necessitates thorough evaluation during primer design, focusing not only on the target sequence but also on potential homologies with other sequences in the genome.
- Annealing Temperature: The temperature at which primers bind to the DNA is critical. Too low of a temperature may allow for nonspecific bindings, while too high could prevent binding altogether. This delicate balance is pivotal for achieving reliable and reproducible results.
- Complex Templates: When using complex genomic DNA as a template, the presence of homologous sequences can further complicate specificity. Variants or related sequences may be inadvertently amplified, making accurate quantification challenging.
Correctly addressing specificity is vital for generating trustworthy results. Researchers must continually assess primer efficiency and target sequence fidelity to minimize ambiguity in their findings.
Contamination Risks
Contamination poses another notable limitation of PCR. While PCR can amplify small amounts of DNA, this very property makes it particularly susceptible to contamination from various sources. Careful handling and rigorous laboratory practices are needed to prevent contamination, as the presence of unintended DNA can lead to erroneous results. Key aspects regarding contamination include:
- Environmental DNA: PCR is highly sensitive; thus, even minute amounts of contaminating DNA from the environment can affect results. Sources include skin cells, hair, or airborne particles. Lab personnel must follow strict protocols to mitigate this risk.
- Carryover Contamination: Samples from previous experiments may inadvertently contaminate subsequent experiments. This is particularly concerning in high-throughput settings, where the risk of carryover can be significant. Implementing separate areas for pre- and post-PCR steps helps to reduce this risk.
- Reagents and Equipment: Contaminated reagents or equipment can introduce error into the experiment. It is advisable to use high-quality, certified reagents and to regularly decontaminate workspaces and tools to uphold the integrity of PCR experiments.
"Awareness of contamination risks is paramount for the reliability of PCR results."
In summary, understanding the limitations of PCR, such as specificity issues and contamination risks, provides a deeper comprehension of the technique. Identifying and mitigating these challenges is critical for achieving accurate and reliable results in molecular biology research. Researchers and professionals alike must remain vigilant as they equip themselves with techniques to manage these limitations effectively.
Future Directions in PCR Technology
The landscape of Polymerase Chain Reaction (PCR) technology is evolving rapidly. This section explores the future directions that continue to enhance the functionality and efficiency of PCR. Understanding these advancements is crucial for students, researchers, and professionals intent on optimizing their molecular biology research or clinical applications.
Innovations in Primer Design
Primer design plays a pivotal role in the success of PCR. In the future, we expect new approaches to improve specificity and amplify the targeted DNA with high fidelity. Recent techniques involve using computational modeling to predict optimal primer pairs. These provide insights into secondary structure and potential dimer formations. Effective primer design can reduce wastage of resources and minimize false positives.
A few innovations include:
- Use of synthetic biology tools: Synthetic biology enables the design of custom primers that can bind selectively to targets, enhancing specificity.
- Incorporating locked nucleic acids (LNA): LNAs increase the melting temperature of primers, improving binding under diverse conditions.
These advancements not only improve the efficiency of PCR but also broadens the scope of its applications.
Integration with Next-Gen Sequencing
The future of PCR is closely tied to next-generation sequencing (NGS). Combining these two powerful technologies can result in more profound insights in genomics. PCR can amplify specific regions of interest before sequencing, resulting in higher overall sensitivity and accuracy in results. This synergy is vital in numerous fields such as clinical diagnostics and environmental testing.
Key considerations in this integration include:
- Improved target enrichment: Amplifying specific genomic regions can lead to better coverage and deeper sequencing data.
- Robustness against contamination: As NGS evolves, integrating more stringent controls in PCR protocols helps mitigate risks associated with contamination.
Integrating PCR with NGS technology makes it possible to analyze large datasets efficiently, facilitating breakthroughs in personalized medicine and genomics.
"The collaboration between PCR and NGS epitomizes the future of genetic research, providing enhanced capabilities that were not possible before."
Closure
The conclusion of this article serves as a pivotal point, summarizing the extensive discussion on Polymerase Chain Reaction (PCR) and its relevance in molecular biology. By reiterating the essential components and applications of PCR, it underscores the transformative nature of this technique in various scientific fields.
Summary of Key Points
Throughout this article, we covered critical aspects of PCR, encapsulating its definition, historical background, and fundamental principles. We explored the intricate steps involved in the PCR process, emphasizing denaturation, annealing, and extension. The applications of PCR were highlighted, showcasing its vital role in clinical diagnostics, genetic research, and forensics. We also discussed significant variations of PCR, including Real-Time PCR and Digital PCR, which illustrate ongoing innovations in this field. In addressing the limitations, we spotlighted specificity issues and contamination risks, offering a balanced view of PCR's potential and constraints.
In summary, PCR is not merely a laboratory technique; it is a driving force behind advancements in genetics, diagnostics, and forensic science.
Importance of Continued Research
The landscape of molecular biology is rapidly changing, making continuous research in PCR technology imperative. Ongoing studies aim to enhance reliability, specificity, and efficiency of PCR, addressing current limitations. Research into innovative primer design and the integration of PCR with next-generation sequencing represents exciting frontiers that could revolutionize genetic analysis. Thus, investment in this area holds the promise of further breakthroughs, expanding our understanding and capabilities within the realms of genetics and molecular diagnostics.
Ultimately, as PCR technology evolves, it will foster new discoveries, enabling scientists to unravel the complexities of genetic material with greater efficacy.