Tunable Quantum Cascade Lasers: Principles and Applications
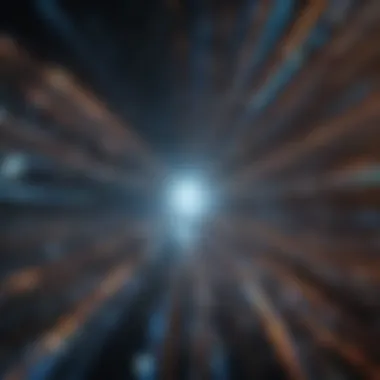
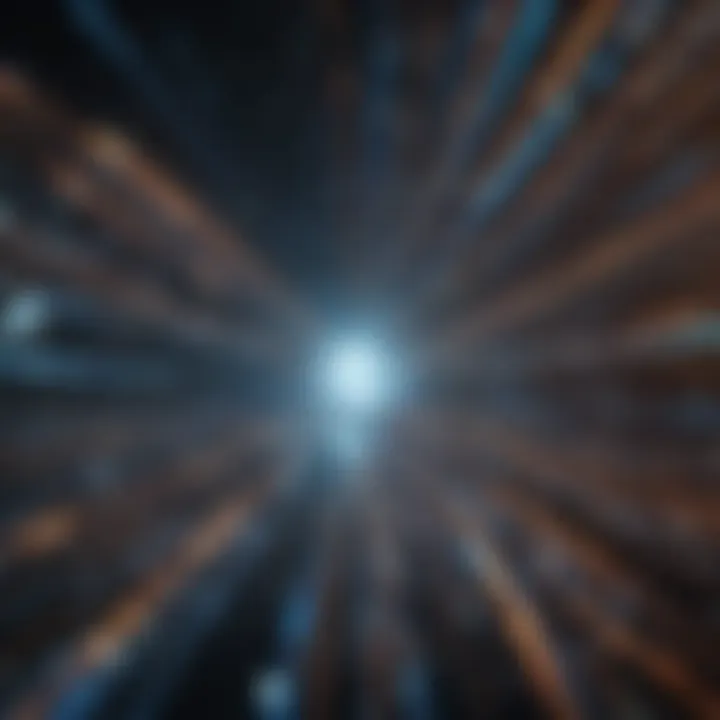
Intro
Tunable quantum cascade lasers (QCLs) have emerged as a groundbreaking technology in laser science, driving advancements across multiple fields. These lasers operate based on the principle of intersubband transitions in quantum wells, allowing for dynamic tunability over a wide wavelength range. This flexibility opens a treasure trove of applications, from sensitive chemical detection to cutting-edge telecommunications.
The significance of QCLs cannot be overstated. They represent a critical intersection of physics, engineering, and practical application, catering to the needs of researchers in various disciplines. Their unique capability to emit light at specific wavelengths makes them invaluable in spectroscopy and sensing applications. For instance, by adjusting the laser, one can target specific molecular vibrations, enabling the detection of trace gases with pinpoint accuracy.
This article endeavors to shed light on the composition and mechanics behind tunable QCLs, offering a thorough understanding of their design variations, fabrication techniques, and practical implications. Whether you are a student, educator, or professional, the complexities surrounding tunable QCLs are sure to provide enticing insights into the forefront of photonics technology.
Prologue to Tunable Quantum Cascade Lasers
Tunable quantum cascade lasers (QCLs) are at the forefront of optical technology, merging the principles of quantum mechanics with photonics to create versatile and high-performance devices. Understanding their role begins with acknowledging their unique capability to emit coherent light across a wide range of wavelengths, making them profoundly significant in many scientific and industrial domains.
In this article, we will guide you through the intricacies of tunable QCLs, dissecting various aspects such as their essential operating principles, unique design variations, and practical applications – essentially providing a roadmap to understanding these advanced optical systems.
Understanding Quantum Cascade Lasers
Quantum cascade lasers represent a revolutionary leap in the laser technology landscape. Unlike traditional lasers, which rely on electron transitions between energy levels in atoms or ions, QCLs operate using engineered semiconductor structures. This allows the emission of light at wavelengths that can be finely tuned according to the needs of the application at hand.
The construction of a QCL involves layers of semiconductor materials that are designed to create quantum wells. When an electrical current is passed through, electrons cascade down these wells, emitting photons at each step. This mechanism not only enables the generation of a rich spectrum of wavelengths but also allows for the fine-tuning of these emissions, promoting their use in various applications ranging from environmental monitoring to medical diagnostics. Such a breadth of application can directly be traced to the basic design and function of QCLs.
Significance of Tunability
The capability to tune the frequency of laser light is what truly sets QCLs apart from many other laser technologies. Unquestionably, tunability enhances the versatility of QCLs in real-world scenarios. Here are some specific benefits:
- Targeted Applications: Tunable QCLs can be adapted to emit wavelengths that correspond to specific molecular transitions, making them particularly useful in spectroscopy. This is vital for chemical analysis since different molecules absorb and emit at distinct wavelengths.
- Environmental Monitoring: In fields such as atmospheric science, having the ability to finely tune a laser allows for precise measurements of trace gases, contributing to our understanding of air quality and climate change.
- Medical Advancements: In the healthcare sector, tunable QCLs facilitate non-invasive diagnostics by allowing researchers and clinicians to identify and analyze biological markers at high sensitivity.
The ability to tune the laser frequency not only enhances functionality but also opens avenues for innovation in fields that rely on precise measurements and interactions with materials at the molecular level.
Overall, this unique tunability aspect amplifies the relevance of quantum cascade lasers, positioning them as pivotal instruments in the modern technological landscape. The knowledge of these foundational principles will set the stage for further discussions on the underlying mechanisms and practical implications of tunable QCLs.
Fundamental Principles of QCLs
Understanding the fundamental principles of Quantum Cascade Lasers (QCLs) roots the discussions around their operational efficacy and applicability. These underlying principles dictate not only how these lasers function but also their potential in various high-tech industries. In this section, we will explore two primary aspects: semiconductor band structure and carrier dynamics. Each plays a vital role in the inherent capabilities of QCLs, informing advancements and practical implementations in fields from telecommunications to environmental sensing.
Semiconductor Band Structure
The semiconductor band structure forms the backbone of Quantum Cascade Lasers. At its core, the band structure defines how electrons exist in a material, determining energy levels and their transitions. For QCLs, this is particularly significant; these lasers are engineered to exploit transitions between subbands in a quantum well rather than the more common electron-hole transitions seen in conventional lasers.
In simpler terms, think of the semiconductor band structure like the layers of an onion. Each layer corresponds to different energy states for electrons. When these layers are engineered thoughtfully, they enable efficient photon generation when electrons transition between these states. This quantum design allows QCLs to emit at specific wavelengths determined by the geometry and material properties.
- Benefits of a well-designed band structure include:
- Tunability across a wide range of wavelengths
- Greater efficiency in generating light
- Potential for high-speed operation under various conditions
Understanding this structure is essential for researchers seeking to design more effective tunable QCLs. Real-world applications, ranging from spectroscopic tools to laser-based sensors, rely heavily on these tailored semiconductor properties.
Carrier Dynamics
Another crucial aspect is carrier dynamics, which refers to the behavior of charge carriers (electrons and holes) within the semiconductor material. This concept is vital in understanding how charge carriers move, recombine, and transition, all of which directly impacts the performance of a QCL.
In the context of QCLs, the design focuses on managing the carrier's ability to jump between levels within the band structure efficiently. This dynamic behavior determines how rapidly the laser can operate and how effectively it can convert electrical energy into optical power.
- Key considerations in carrier dynamics include:
- Recombination processes: Efficient photon generation necessitates appropriate transitions without excessive recombination losing the energy prematurely.
- Carrier scattering: The movement of carriers can be impeded by various scattering processes, influencing overall efficiency and speed.
In a nutshell, mastering these aspects of carrier dynamics allows for fine-tuning of laser output in terms of both efficiency and operational range. For any researcher or engineer working with QCLs, comprehending the interplay between band structure and carrier dynamics is paramount.
"The effectiveness of QCLs is intrinsically tied to the meticulous orchestration of semiconductor band structure and carrier dynamics; both are foundational to the technology's allure in advanced applications."
Through understanding these fundamental principles, the stage is set for innovative designs and applications of tunable Quantum Cascade Lasers. With insights into how alterations at the quantum level yield significant practical benefits, researchers are equipped to push the boundaries of what’s possible in laser technology.
Mechanism of Tunable Emission
Understanding the mechanisms that allow tunable emission in quantum cascade lasers (QCLs) gives key insights into their operational capabilities. At the heart of this technology lies the ability to adjust the emission wavelength, which holds significant implications for diverse applications such as spectroscopic analysis and chemical detection. Tunability not only enhances flexibility but also boosts a QCL’s potential utility across various fields. Therefore, this section sheds light on two primary tuning mechanisms: electro-optic control and temperature-dependent tuning.
Electro-Optic Control
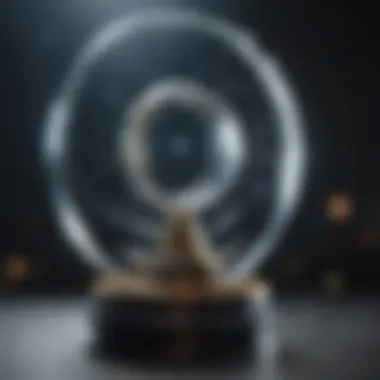
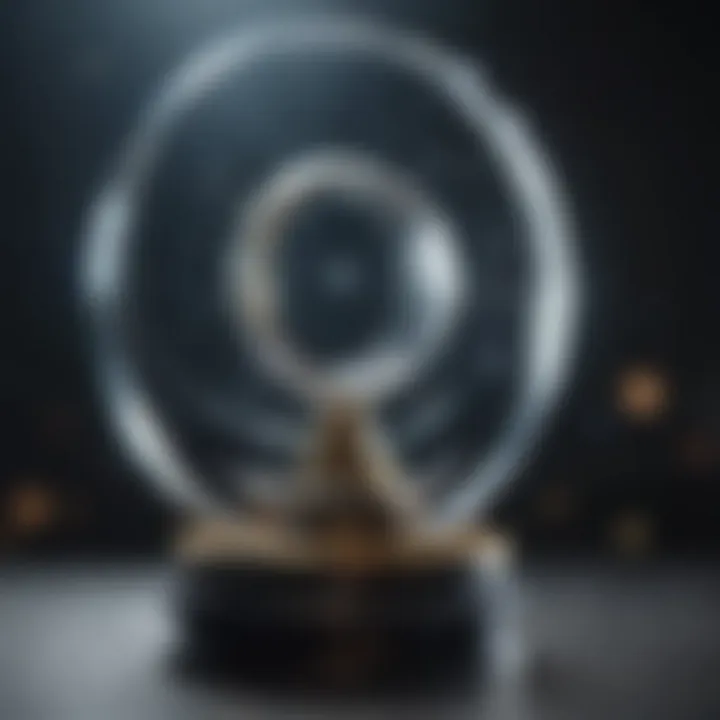
Electro-optic control is a fundamental mechanism that enables the tuning of wavelength in quantum cascade lasers by applying an external electric field. This approach relies on the phenomenon known as the Franz-Keldysh effect, which effectively gets the electrons moving in the conduction band. By varying the electric field, the band structure of the semiconductor can be modified.
Consider a situation where a specific laser emission is required for a precise measurement in a laboratory setting. By using electro-optic control, researchers can fine-tune the output to match the exact absorption characteristics of the target substance. This efficient adjustment allows for minimal interference during measurements, enhancing accuracy.
Advantages of Electro-Optic Control:
- Quick Wavelength Adjustment: Users can rapidly change the output wavelength, which is highly beneficial in dynamic environments.
- High Precision: The control mechanism allows for precise adjustments, leading to enhanced measurement accuracy.
- Versatility: This method can be integrated into various laser designs and applications, making it widely applicable across different fields.
However, incorporating electro-optic control does introduce some challenges. Devices must manage the heat that is produced during operation and ensure that the components maintain their integrity over many cycles.
Temperature-Dependent Tuning
Temperature-dependent tuning relies on the fact that changes in temperature affect the refractive index and relationship between energy levels in the quantum well structures of QCLs. As temperature fluctuates, the energy states shift, allowing for variation in the emitted wavelength. This tuning approach is especially prevalent where broad spectral range is necessary.
Imagine a scenario where a QCL is utilized to identify trace gases in environmental monitoring. By adjusting the operational temperature, it’s possible to broaden the wavelength range, thereby enhancing detection capabilities for multiple substances. This method's reliability renders it a popular choice in practical applications.
Key Points of Temperature-Dependent Tuning:
- Broadened Spectral Range: This mechanism permits a wider array of wavelengths to be accessed, which facilitates diverse applications.
- Easy Integration: Temperature tuning can be seamlessly included in many existing setups because it often requires simpler adjustments than other methods.
- Simplicity in Design: Without the need for complex electronic systems, temperature-dependent tuning can keep QCL designs more straightforward.
Despite its advantages, tuning by temperature control can face challenges. Changes in external temperature may lead to unwanted fluctuations, and thus consistent operational environments must be maintained.
"The ability to finely tune the wavelength in quantum cascade lasers enables researchers to adapt their methods and technologies for an array of real-world applications, showing just how enthralling the science behind these lasers can be."
Design Variations of Tunable QCLs
The design of tunable quantum cascade lasers (QCLs) plays a crucial role in the performance and applicability of these devices. The ability to tailor their emission characteristics through various design variations not only enhances their functionality but also broadens their use in an array of scientific fields. In this section, we will delve into two prominent design variations of tunable QCLs: distributed feedback QCLs and broadly tunable QCLs, highlighting their unique features, benefits, and the considerations that come with each approach.
Distributed Feedback QCLs
Distributed feedback QCLs (DFB QCLs) represent a significant evolution in lasing technology. Characterized by a periodic structure, these lasers incorporate an optical feedback mechanism that is integral to their operation. The uniqueness of DFB QCLs lies in their ability to produce a single longitudinal mode of laser light, thus minimizing spectral linewidth. This property is particularly important in applications where high resolution is required, such as in spectroscopy.
- Improved Mode Control: The periodic waveguide structure of DFB QCLs enhances the control over the emitted wavelength. With careful design, it's possible to select specific wavelengths by adjusting the grating period, allowing researchers to tailor lasers for distinct applications.
- Applications: Due to their narrow emission linewidths, DFB QCLs are extensively used in high-resolution spectroscopic measurements. They are effective in environmental monitoring, remote sensing, and medical diagnostics, where precise wavelength control can make a significant difference.
- Considerations: Crafting an efficient DFB QCL requires a delicate balance between performance and complexity. The processing to create the grating can be intricate and must adhere to high precision standards to avoid affecting the laser’s operational reliability.
“The brilliance of DFB QCLs lies in their ability to achieve exceptional spectral purity, which opens doors to numerous scientific applications.”
Broadly Tunable QCLs
Broadly tunable QCLs stand out due to their flexibility in emission wavelength. Unlike DFB QCLs that favor a narrow wavelength range, broadly tunable QCLs are designed to cover a wide spectrum, making them versatile tools in various scientific applications. The broad tunability is mainly achieved through innovative design techniques.
- Wavelength Coverage: Broadly tunable QCLs can emit over several hundred nanometers at a time, which allows them to be used in applications where multiple wavelengths are necessary. This flexibility is vital in fields like chemical sensing, where detecting a range of specific absorption lines is needed for accurate measurements.
- Dynamic Tuning: Many broadly tunable QCLs employ nonlinear optical processes, such as parametric oscillation, enabling real-time adjustments to the output wavelength. This feature makes them suitable for labs requiring rapid wavelength switching, often crucial in research settings.
- Considerations: Although their versatility is a big advantage, broadly tunable QCLs also face challenges. Managing thermal properties is key since tunability can affect efficiency and scalability, making the manufacturing process complex. Additionally, ensuring that the laser maintains a high level of output power across the tuning range can be challenging.
Fabrication Techniques
The fabrication of tunable quantum cascade lasers (QCLs) is a critical aspect that directly influences their performance, efficiency, and applicability in various fields. Understanding the techniques involved helps to bridge the gap between theoretical designs and practical devices, enabling advancements in technology. Each step in the fabrication process plays a pivotal role in achieving the desired characteristics of the laser, such as tunability, power output, and operational stability. Without proper fabrication methods, the potential of QCLs would remain largely untapped.
Molecular Beam Epitaxy
Molecular Beam Epitaxy (MBE) is a widely recognized method for growing semiconductor materials in a controlled environment. It allows for the creation of high-quality heterostructures essential for QCLs. The technique enables precise control over the composition and thickness of the layers, which can be crucial, as even slight variances can lead to significant performance differences.
In MBE, atoms or molecules are deposited onto a substrate in a vacuum. This environment minimizes contamination and enhances the uniformity of the materials. Some benefits of MBE include:
- High Quality: Layers formed are exceptionally pure and defect-free, essential for effective lasing.
- Control: The growth rate and temperature can be finely tuned, leading to reproducible results.
- Flexibility: MBE accommodates a wide variety of materials, making it adaptable for different applications.
The precision in layer thickness is important for achieving the desired optical and electronic properties, ensuring the laser operates at specific wavelengths as required. The ability to customize the materials and build complex structures makes MBE indispensable in the fabrication of tunable QCLs.
Characterization Methods
Once the QCLs are fabricated, it is crucial to assess their performance through characterization methods. Characterization provides insights into the operational capabilities and limitations of the lasers. Various techniques can be employed, each serving a unique purpose in evaluating the devices. Some of the key characterization methods include:
- Spectroscopy: This allows researchers to examine the emission spectra of QCLs, helping to ensure that they align with expectations for tunability and output.
- Temperature Measurement: Understanding how temperature affects the laser's behavior is vital. Techniques like thermography help in monitoring heat distribution, which is essential for performance optimization.
- Electrical Characterization: Assessing parameters like current-voltage characteristics gives insights into efficiency and operational stability.
"Characterization not only verifies the success of the fabrication process but also guides future designs and improvements."
Each characterization method contributes essential data that assists in understanding how different fabrication techniques influence the performance of the QCLs. It’s an iterative process that promotes constant refinement in both design and manufacturing approaches.
Ultimately, mastering these fabrication techniques and using appropriate characterization methods serve not just to advance the current state of tunable quantum cascade lasers, but to open new avenues for research and application across diverse sectors.
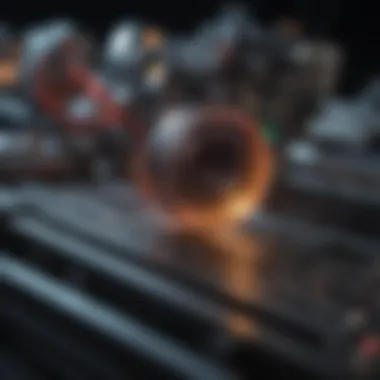
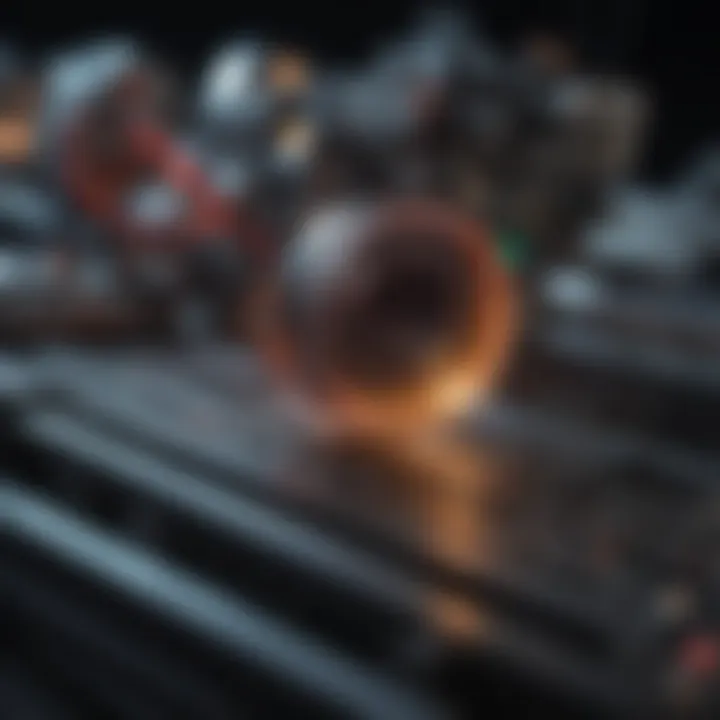
Performance Optimization
In the realm of tunable quantum cascade lasers, achieving optimal performance is critical. These lasers stand out because of their ability to precisely target various wavelengths, but to fulfill their potential, they must exhibit efficiency and reliability. Optimizing performance touches upon multiple facets of the laser's operation, encompassing energy usage, output power, and stability under varying conditions. Consequently, how well these lasers optimize can significantly influence their effectiveness in applications ranging from chemical analysis to telecommunications. Understanding and improving performance is not just an option; it's a necessity for advancing technologies where precision and efficiency are paramount.
Efficiency Improvements
Efficiency is one of the cornerstones of any high-functioning laser system, and tunable QCLs are no exception. The improvement of the overall efficiency of these lasers can derive from several factors:
- Material Quality: Utilizing high-purity materials leads to fewer defects. Defects tend to scatter electrons, reducing the overall efficiency, so materials must be as flawless as possible.
- Optimized Layer Structure: The band structure of QCLs plays a pivotal role. Engineers often adjust the quantum well widths or even the doping concentrations to fine-tune the laser’s efficiency. Essentially, a well-structured design allows electrons to transition more effectively, thereby generating more light.
- Heat Management: Efficient cooling systems help maintain optimal operating temperatures. Overheating can diminish performance, so employing advanced thermal management techniques, like microchannel coolers, can improve efficiency.
When taken together, these aspects not only enhance laser operation but also inform future designs aimed at pushing the boundaries of what tunable QCLs can achieve.
Noise Reduction Techniques
In lasers, noise can be a major performance bottleneck. For tunable quantum cascade lasers, managing this unwanted interference is crucial for applications that demand precision, such as spectroscopy and chemical sensing. Here are some standards methods to reduce noise levels:
- Feedback Stabilization: Implementing optical feedback control can greatly help in minimizing fluctuations. By incorporating a feedback loop, the output can be stabilized, aiding the laser to maintain a consistent wavelength in the presence of external disturbances.
- Layer Engineering: Tailoring the thickness of active regions or varying the number of periods in the quantum structure can lead to improved uniformity in output power, thus reducing noise.
- Careful Placement of Components: Choosing the right environment for your setup can mitigate external disturbances. Like in general acoustics, reducing vibrational effects within the experimental environment or even during deployment can substantially improve laser stability.
As emphasized, the factors affecting noise can be subtle but crucial in ensuring the efficiency and reliability of QCLs.
Applications of Tunable QCLs
Tunable quantum cascade lasers (QCLs) are not just a bunch of fancy jargon tossed around in technology discussions. They have become pivotal in several fields due to their unique ability to precisely control the wavelength of light emitted. This tunability allows them to excel in various applications, particularly in spectroscopy, chemical sensing, and telecommunications. Each of these areas benefits significantly from the engineering marvel that tunable QCLs represent.
Spectroscopic Techniques
One of the most impressive applications of tunable QCLs lies in their role in spectroscopic techniques. Spectroscopy, at its core, is all about studying the interaction between light and matter, and tunable QCLs provide a versatile tool for scientists to analyze materials at molecular levels. Unlike conventional lasers that might emit a fixed wavelength, tunable QCLs can be adjusted to probe specific molecular transitions, which means they can detect a wider range of substances.
The impact is huge when considering the nuances of a substance's composition. They allow for precise measurements of absorption spectra, helping researchers characterize materials and identify chemical compounds in various environments, from atmospheric studies to biomedical analysis. This flexibility in wavelength can lead to more accurate results in techniques like Fourier-transform spectroscopy or photoacoustic spectroscopy.
"Tunable QCLs revolutionize spectroscopic approaches, enabling unprecedented insight into molecular characteristics."
Furthermore, the adaptability of tunable QCLs is essential in remote sensing and environmental monitoring. These lasers can be deployed over vast areas to detect pollutants or greenhouse gases, offering insights that were either difficult or impossible to gather before.
Chemical Sensing
Another realm where tunable QCLs shine is chemical sensing. The laser’s ability to fine-tune its emission wavelength allows for the identification and quantification of various gases and vapors in real-time. In industries like petrochemicals and food safety, this capability cannot be overstated.
For instance, the infrared wavelength range covered by QCLs is particularly conducive to detecting many chemical species, such as carbon dioxide and methane, at trace levels. This trait is beneficial in applications ranging from industrial safety to environmental management.
Some practical instances include:
- Smart sensors used for leak detection in pipelines.
- Portable analyzers monitoring air quality in urban environments.
- In-situ measurements for determining contaminants in food and water supplies.
The speed and sensitivity of tunable QCLs give them a significant edge over traditional methods, providing quicker responses and the ability to operate in complex environments.
Telecommunications
As we venture into the telecommunication sector, the importance of tunable QCLs becomes even more evident. With data demands skyrocketing in today's world, innovative solutions for optical communication are crucial. Tunable QCLs can serve as sources that transmit data over fiber-optic networks, leveraging their capacity to rapidly and efficiently adjust wavelengths.
Their application in optical frequency combs facilitates advances in high-capacity data transmission. Moreover, the ability to rapidly switch wavelengths aids in wavelength division multiplexing (WDM), significantly increasing the overall bandwidth of communication channels.
The potential role of tunable QCLs in supporting the next generation of wireless technology cannot be overemphasized, particularly in providing faster and more reliable mobile communications, which are essential as we move towards advanced concepts like the Internet of Things (IoT).
In essence, the applications of tunable QCLs extend far beyond obvious uses in laser technology. They are reshaping how we understand and interact with the world at a molecular level, offering new techniques for research and development across multiple sectors, while pushing the boundaries of existing technologies.
Recent Advancements in QCL Technology
Recent strides in quantum cascade laser technology mark a pivotal chapter in its development. With an ever-growing demand for precision and adaptability in lasers, these advancements open numerous doors not just in research and industry but also in applications that were once thought to be out of reach. The focus on optimizing performance through material innovations and device architectures is crucial. Below, we break down the latest trends that are reshaping the landscape of tunable QCLs.
New Material Developments
The advent of new materials plays a significant role in enhancing the performance of quantum cascade lasers. Researchers are investigating alternative semiconductor materials beyond the traditional InGaAs, like GaN and AlInGaAs. These materials can lead to improved thermal stability and a wider range of tunability. For example, using GaN allows for higher temperature operation without a drop in performance, thereby expanding the potential applications of QCLs in harsh environments.
Another noteworthy advancement is the burgeoning interest in nanostructured materials. By incorporating nanowires and quantum dots, these structures offer tailored emission characteristics which are not possible with bulk materials. This promotes better control over the laser's wavelength and thus provides efficiencies that can benefit various spectroscopic applications.
Additionally, the integration of 2D materials such as graphene is garnering attention. Interestingly, graphene's unique electronic properties may allow for some groundbreaking modifications in how the lasers operate. For instance, it can be utilized as an efficient heat sink, reducing operating temperatures.
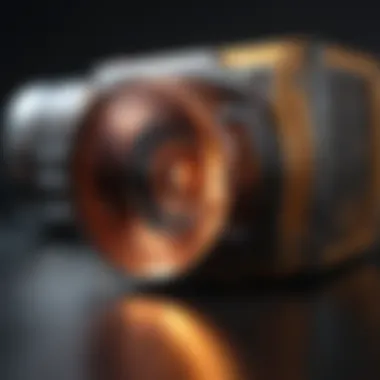
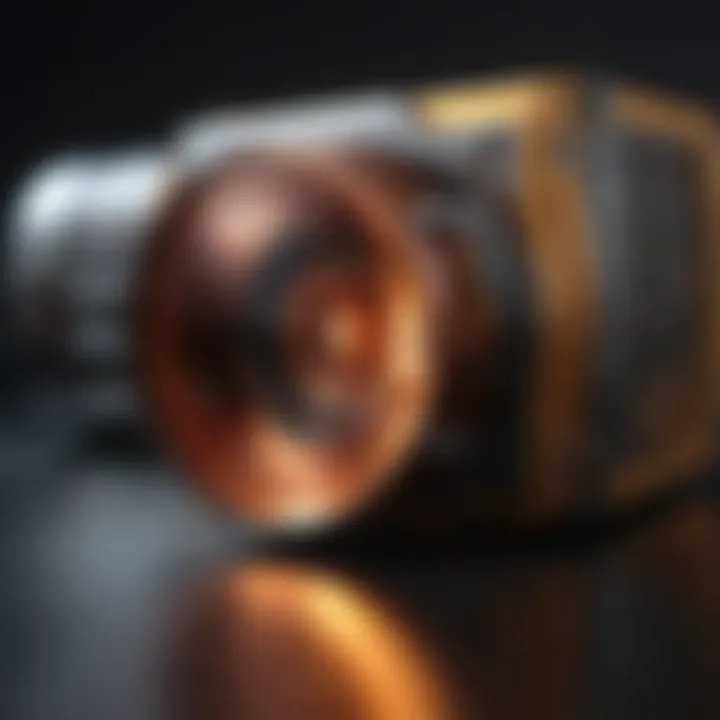
Innovative Device Architectures
Beyond materials, the architecture of the devices themselves has also seen revolutionary changes. Designers are focusing on compact and integrated systems that can be easily incorporated into existing technologies. One such trend is the development of chip-scale tunable QCLs, which promise smaller form factors while maintaining performance. These micro-sized lasers can fit into portable devices that require high sensitivity for monitoring applications, thus expanding the market reach.
The introduction of optical feedback mechanisms further enhances output stability and tunability. By utilizing integrated Bragg mirrors, photonic crystals, or even custom-designed resonator structures, these architectures can stabilize the laser output against environmental fluctuations and allow for rapid wavelength tuning. Such systems are proving invaluable in areas like chemical sensing and telecommunications, where precision is paramount.
"New architectures and materials are driving tunable QCLs to the forefront of laser technology, pushing boundaries that have defined the industry for decades."
The evolution of device architecture not only enriches laser functionality but also their compatibility with other technologies.
In summary, these advancements signify a broadening horizon for tunable quantum cascade lasers, amplifying their impact across various sectors with newly engineered materials and innovative designs. By leveraging new developments in materials science and engineering techniques, researchers and manufacturers are well on their way to a future where tunable QCLs become integral to modern technology.
Challenges and Limitations
In the swiftly evolving landscape of tunable quantum cascade lasers (QCLs), understanding the challenges and limitations is not just an academic exercise; it is an essential element in advancing the technology's capabilities. The importance of this topic lies in addressing critical factors that could hinder development and practical applications while simultaneously outlining potential pathways for innovation.
Heat Management Issues
One of the foremost challenges associated with the usage of tunable QCLs is managing heat efficiently. As these lasers operate, they can generate considerable amounts of heat, which may lead to performance degradation if not adequately handled. Improper thermal management can affect not only the laser's efficiency but also its lifespan. Excessive heat can induce shifts in the semiconductor band structure, ultimately affecting output wavelength and causing instability in laser operation.
- Impact on Efficiency: The efficiency of QCLs can plummet if heat isn't handled properly. Higher temperatures can lead to increased thresholds for lasing, thus demanding more power input.
- Cooling Solutions: Various cooling solutions exist, such as using thermoelectric devices or advanced liquid cooling systems. Implementing effective heat dissipation strategies, such as heat sinks and good thermal contact layers, is crucial for maintaining optimal performance.
Integration with Other Technologies
Another facet of the challenges surrounding tunable QCLs is their integration with existing technologies. While QCLs hold great potential in applications ranging from spectroscopy to chemical sensing, they can't operate in isolation. The integration process can be complex and requires a delicate balance between interfacing with multiple systems and maintaining laser performance.
- Compatibility Issues: Different technologies often have unique requirements and standards. This can pose hurdles in achieving seamless operation. For instance, embedding these lasers into existing telecommunications systems might necessitate adaptations in signal processing architecture.
- Multidisciplinary Collaboration: Collaborating across various domains, such as materials science, electronics, and optics, is essential to develop robust integration solutions. Building custom interfaces or modulation techniques can enhance compatibility while expanding application capabilities.
The journey toward fully realizing the potential of tunable QCLs will require tackling these challenges head-on, offering a fertile ground for research and development.
Addressing these challenges can unveil new avenues for performance enhancement and broaden the applicability of tunable QCLs. Through dedicated efforts in both heat management and technology integration, the future of QCL development looks promising.
The Future of Tunable QCLs
The realm of tunable quantum cascade lasers (QCLs) stands on the brink of significant transformations, intertwining advancement with innovation. As science and technology march forward, the future of QCLs is not just about improvements, but about reshaping entire fields of research and application. The importance of this topic within the article lies in understanding how emerging trends can elevate performance and functionality, thereby unlocking new possibilities. With continuous enhancements in tunability, efficiency, and versatility, the landscape of laser technology is bound to change dramatically.
Potential Research Directions
Key areas are ripe for exploration, including:
- New Material Development: Research into novel semiconductor materials can drastically enhance the performance of QCLs. Investigating alternatives to traditional materials could lead to better heat management and tuning capabilities.
- Integration with Quantum Technologies: As quantum computing and related technologies progress, there's substantial potential for integrating QCLs with these systems. Real-time data processing applications could benefit immensely from this!
- Advanced Manufacturing Techniques: Innovations in fabrication, such as three-dimensional printing and nano-fabrication, could provide more precise control over laser parameters, paving the way for higher quality and more complex designs.
- Broadened Operating Ranges: Expanding the tuning range of QCLs is another avenue for future research. This may involve re-engineering the active layers and optimizing carrier dynamics to broaden spectral coverage.
Through these avenues, the ongoing research on QCLs may lead to significant breakthroughs that could change their application scope and operational efficiency.
Commercial Opportunities
The market potential for tunable QCLs is staggering. Applications stretch from environmental monitoring to medical diagnostics, showcasing their versatility and adaptability. Consider:
- Sensing Technologies: QCLs can be commercially exploited in environmental sensing for gases and pollutants. Their sensitivity can offer real-time monitoring solutions, which are a necessity for regulatory compliance.
- Healthcare Applications: Utilization of QCLs in non-invasive medical devices could enhance diagnostic processes. For instance, early detection of diseases through breath analysis is becoming a palpable commercial avenue.
- Telecommunications: The advent and expansion of fiber optics have led to a burgeoning demand for high-speed communication technologies where tunable QCLs could play a pivotal role in enhancing bandwidth and reducing latency.
- Industrial Use: In manufacturing, QCLs can facilitate more efficient laser processing techniques that minimize waste while maximizing output.
By capitalizing on these opportunities, the future of tunable QCLs holds the promise not just of new products and services, but entire industries could be transformed due to their key functionalities.
"Bringing QCL technology from the lab to real-world applications defines the future of how we approach science and technology."
With the right focus on research and commercialization, tunable quantum cascade lasers could very well become a cornerstone in multiple sectors, driving innovation and significant advancements.
End
The conclusion is not merely a summary; it's the capstone of a comprehensive journey through the realm of tunable quantum cascade lasers. This section encapsulates the key insights and emphasizes the relevance of QCL technology, which is crucial for researchers and practitioners. Understanding why tunable QCLs matter can lead to further innovations in various scientific disciplines, and this warrants careful consideration.
Summary of Key Points
In this article, we have traversed various facets of tunable quantum cascade lasers. Here’s a succinct recap of the main points discussed:
- Fundamental Principles: We delved into the semiconductor band structures and carrier dynamics, laying the groundwork for understanding how QCLs operate.
- Mechanisms of Tunable Emission: The article dissected electro-optic control and temperature-dependent tuning, interpreting the tuning mechanisms essential for applications.
- Design Variations: We explored distributed feedback QCLs and their broader tuning counterparts, illuminating the innovative approaches in device design.
- Fabrication Techniques: Molecular beam epitaxy and characterization methods were elucidated, providing insight into the construction of these sophisticated lasers.
- Performance Optimization: The discussions on efficiency and noise reduction techniques spotlighted ongoing efforts to enhance these lasers’ performance.
- Applications: Diverse applications, including spectroscopic techniques and telecommunications, showcased the broad utility of tunable QCLs in real-world scenarios.
- Challenges and Limitations: The sections dedicated to heat management issues and the integration of QCLs with other technologies outlined practical hurdles faced by researchers.
- Future Perspectives: Potential research directions and commercial opportunities were presented, framing the future landscape for QCL technology.
This summary underscores the intricate web of knowledge that surrounds tunable QCLs and their significance in pushing the boundaries of science and engineering.
Implications for the Field
The implications of our findings resonate across multiple disciplines. Tunable quantum cascade lasers are pivotal not only in advancing fundamental research but also in bridging gaps between theory and practical application. Here are some of the key implications:
- Enabling Precision in Measurement: The tunability aspect allows for extremely precise measurements, essential in spectroscopy. Researchers can detect minute changes in chemical compositions or physical states with remarkable accuracy.
- Enhancing Sensitivity in Chemical Sensing: With improved sensitivity, applications in environmental monitoring and industrial processes may transform how we manage resources and assess environmental impacts.
- Driving Innovations in Telecommunications: As communication systems evolve, tunable QCLs offer pathways to better signal integrity and lower interference, which can accelerate the development of next-generation telecommunication infrastructures.
- Informing Material Science: The research surrounding QCLs, especially regarding new material developments, could lead to discoveries that benefit other technologies, such as electronics and photonics.
- Promoting Interdisciplinary Collaboration: The complexity of challenges associated with QCLs necessitates collaboration across multiple fields, driving partnerships between physicists, engineers, and chemists. This interconnected approach can foster innovation and stimulate advancements in related technologies.