The Rule of Protein: Insights into Dynamics and Research
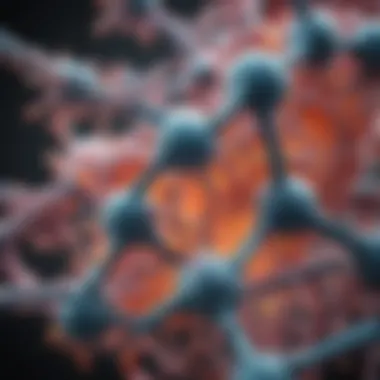
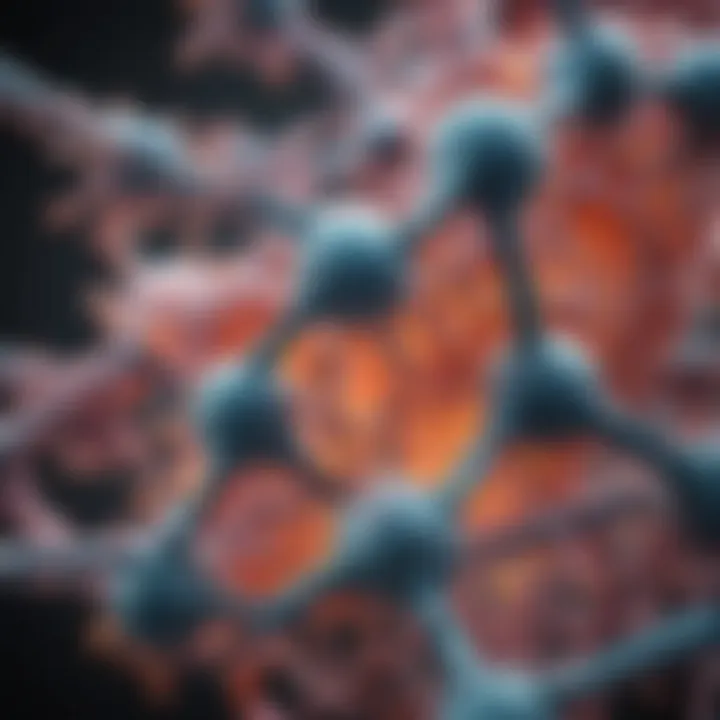
Intro
Proteins are indispensable macromolecules, playing a central role in nearly all biological processes. Their dynamic nature allows them to perform various functions, from catalyzing biochemical reactions to facilitating cellular communication. Understanding the fundamentals of protein dynamics can unlock insights across numerous scientific domains, including biochemistry, molecular biology, and biotechnology.
In recent years, extensive research has revealed the multifaceted roles proteins play not only in living organisms but also in technological advancements. As we explore the world of proteins, we will delve deep into their structural characteristics, the mechanisms that govern their actions, and the implications of recent findings. This comprehensive examination aims to highlight the significance of proteins in both foundational science and practical applications.
Methodologies
In the quest to understand proteins, researchers employ various methodologies that encompass multiple disciplines. These approaches allow scientists to dissect the complexity of proteins at molecular levels.
Description of Research Techniques
A variety of techniques are utilized to study proteins:
- X-ray Crystallography: This technique provides detailed information on the atomic structure of proteins by interpreting diffraction patterns from crystallized samples.
- Nuclear Magnetic Resonance (NMR) Spectroscopy: NMR offers insights into the structure and dynamics of proteins in solution, providing an understanding of their functional state.
- Cryo-Electron Microscopy: This method enables visualization of proteins in their native state at a near-atomic resolution, crucial for studying large complexes.
- Mass Spectrometry: Used for analyzing protein structures, mass spectrometry can identify and quantify proteins in complex mixtures.
These techniques complement each other and together contribute to a comprehensive understanding of protein structure and function.
Tools and Technologies Used
The enhancement of protein studies significantly relies on advanced tools and technologies:
- Bioinformatics Software: Tools like BLAST and CLUSTAL are essential for sequence alignment and protein modeling.
- Protein Expression Systems: Yeast, bacteria, and mammalian cells are used to express recombinant proteins, which helps in studying their functions.
- Laboratory Instruments: Devices such as spectrophotometers and centrifuges facilitate various experimental procedures.
These technologies streamline the research process, enabling more precise investigation into protein functionalities.
Discussion
Research in protein dynamics has evolved significantly, and it is important to contextualize current findings with past studies.
Comparison with Previous Research
The journey of protein research has seen trends shift over decades. Earlier studies primarily focused on protein isolation and basic categorization. In contrast, modern research emphasizes detailed interactions, functional mechanisms, and implications of mutations. For instance, the discovery of the role of chaperones in protein folding has built upon previous knowledge of basic structural roles of proteins, significantly advancing our understanding of cellular biology.
Theoretical Implications
The advancements in protein research reveal substantial theoretical implications. The concerted movement towards integrating computational models with experimental data contributes to the development of theories regarding protein behavior in diverse environments. As the field progresses, these theories enhance predictive capabilities that can be used across disciplines, from designing new drugs to developing biomaterials.
The understanding of proteins influences advancements in medicine, environmental science, and biotechnology, underscoring their crucial role in scientific research.
Through this exploration, we not only gain insights into the fundamentals of protein dynamics but also illuminate the path for future research directions. The intricacies of protein functions continue to inspire scientific inquiry, revealing the complexities of life itself.
Understanding Protein Structure
Protein structure is foundational to understanding the myriad functions that proteins fulfill in biological systems. Grasping how proteins are organized and how this relates to their functionality is crucial, especially in scientific research. By comprehending the various levels of protein structure, researchers can infer how alterations to this structure may impact overall protein function and, subsequently, cellular behaviors.
Basic Protein Composition
Proteins are primarily composed of amino acids, which are organic compounds made of carbon, hydrogen, oxygen, nitrogen, and sometimes sulfur. There are twenty standard amino acids that serve as the building blocks of proteins. The specific sequence and composition of these amino acids lead to the vast diversity of proteins found in nature. Each amino acid has a unique side chain, that determines its properties and roles in protein structures. The correct assembly of these amino acids into proteins is essential for maintaining proper cellular function and overall biological health.
Levels of Protein Structure
Primary Structure
The primary structure of a protein refers to the linear sequence of amino acids connected by peptide bonds. This sequence is determined by the genetic code, and any alteration in the sequence can lead to significant changes in protein function. One key characteristic of primary structure is its uniqueness; no two proteins have identical sequences. This attribute helps researchers identify proteins within various biological systems. However, the primary structure alone does not determine the protein's final shape or function, making it a crucial yet limited starting point for understanding protein dynamics.
Secondary Structure
Secondary structure pertains to local folding patterns within the protein, typically in the form of alpha helices and beta sheets. These structures arise from hydrogen bonding between the backbone of the amino acids. The presence of these patterns enhances the protein's stability. The key characteristic are the predictable shapes these structures form, which provide insight into the overall protein architecture. Still, secondary structure may not be entirely stable on its own; it requires further folding into tertiary or quaternary structures to gain proper function.
Tertiary Structure
The tertiary structure describes the complete three-dimensional shape of a single protein molecule. This structure results from interactions among the side chains of amino acids, such as hydrophobic interactions, ionic bonds, and disulfide bridges. Understanding tertiary structure is beneficial because it provides insights into how a protein functions in a cellular context. Its unique feature is that the folding can be influenced by environmental factors like pH and temperature. A disadvantage is that misfolding can lead to loss of function and diseases, highlighting the necessity for correct tertiary structure.
Quaternary Structure
Quaternary structure involves the assembly of multiple polypeptide chains into a larger functional unit. This structural level is particularly important for proteins that operate as complexes, such as hemoglobin, which consists of four subunits. A key characteristic of quaternary structure is its ability to create a functional protein from non-functional subunits, enabling various biological activities. Its unique feature, the cooperativity between subunits, allows for sophisticated regulatory mechanisms in enzyme activity or binding. While quaternary interactions promote functional diversity, they can also complicate the study of protein functionality due to the dependence on multiple protein variants.
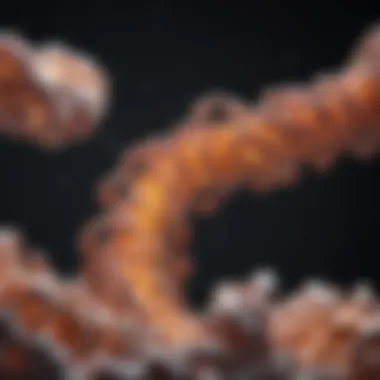
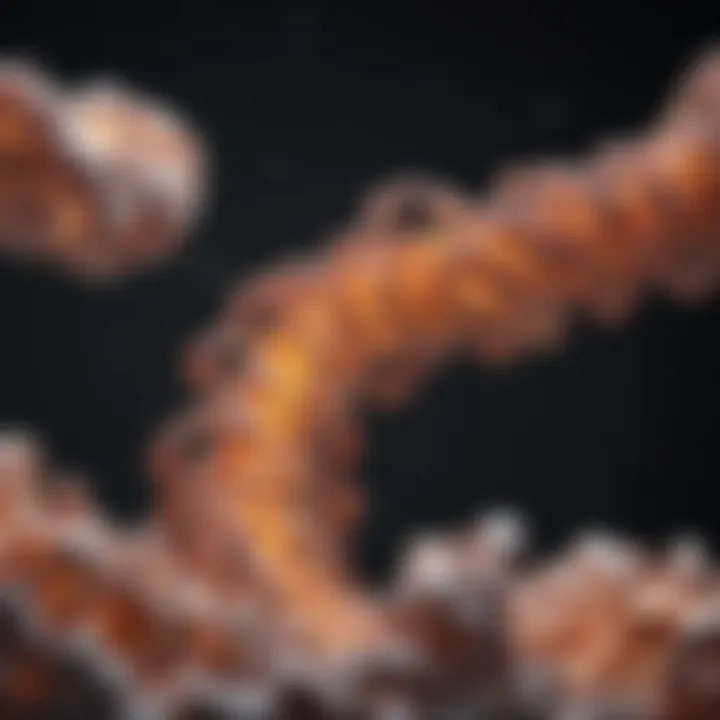
Common Protein Folding Patterns
Proteins fold into specific patterns that are essential for their function. Common patterns such as loops, helices, and sheets often contribute to a protein's stability and function. These folding patterns are not arbitrary; they result from the unique properties of the amino acids within the protein. Understanding these patterns can assist scientists in predicting protein behavior in various conditions, thus aiding in the development of therapeutic strategies and biotechnological applications.
The study of protein structure is integral to advancing our knowledge in biochemistry and molecular biology. Understanding how proteins are structured directly informs their function and the consequences of dysfunction.
Protein Functions in Biological Systems
Proteins serve as the workhorses of the cellular environment, orchestrating a vast array of biological functions. Understanding the functions of proteins in biological systems is crucial for comprehending how life operates at a cellular level. Proteins are involved in nearly every biological process, making their study relevant not only in biology but also in medicine, biotechnology, and environmental science. Each type of protein plays a unique role, contributing to various physiological processes and overall homeostasis.
Enzymatic Proteins
Enzymatic proteins, or enzymes, are catalysts that speed up chemical reactions within cells. They lower activation energies for reactions, thus facilitating metabolic processes. Enzymes are highly specific, often interacting with particular substrates to yield specific products. For example, lactase helps in the breakdown of lactose into glucose and galactose. This specificity allows cellular processes to be efficiently regulated, impacting metabolism and cellular energy levels. The mechanisms of enzymatic action can also be influenced by temperature, pH, and the concentration of substrates, which is important for effective biological function.
Structural Proteins
Structural proteins provide support, shape, and strength to cells and tissues. Collagen, for example, forms the structural framework in connective tissues, while keratin serves a protective role in hair and nails. These proteins are effective due to their stable structures, which withstand various forces. The composition and organization of structural proteins are essential for maintaining the integrity of cells and the overall organism. Understanding these components is vital not only for basic biological research but also for applications in regenerative medicine, where repairing or replacing damaged tissues is needed.
Transport Proteins
Transport proteins are responsible for the movement of molecules across cellular membranes. Hemoglobin, a well-known transport protein, carries oxygen from the lungs to tissues and returns carbon dioxide back to the lungs. Membrane proteins, such as aquaporins, facilitate the transport of water and ions, crucial for maintaining cellular homeostasis. These proteins are fundamental in both nutrient uptake and waste removal within cells. An understanding of transport mechanisms underpins many physiological processes and is key for therapeutic interventions in conditions such as anemia and dehydration.
Regulatory Proteins
Regulatory proteins control and modulate biological processes, playing an essential role in signaling pathways and gene expression. Transcription factors, for instance, bind to specific DNA sequences, regulating the transcription of genes. These proteins act as switches that can turn processes on or off in response to internal or external stimuli. Regulatory mechanisms can be intricate, often involving feedback loops that ensure the stability of cellular functions. Research into these proteins is critical for developing targeted therapies in complex diseases like cancer, where regulatory pathways may become dysregulated.
Understanding the distinct functions of proteins not only illuminates how life sustains itself on a cellular level but also provides therapeutic insights, opening avenues for advancements in healthcare and biotechnology.
The Importance of Proteins in Cellular Processes
Proteins are essential biomolecules that play vital roles in numerous cellular processes. Understanding these roles is crucial in various fields, from molecular biology to biotechnology. Proteins serve as the machinery of the cell, driving metabolic pathways, transmitting signals, and facilitating the synthesis of other molecules. Their importance is underscored by the fact that nearly every cellular function relies on proteins in some manner.
Role in Metabolism
Proteins are key participants in the metabolic pathways that sustain life. Enzymatic proteins, which function as catalysts, accelerate biochemical reactions necessary for metabolism. Without enzymes, these reactions would occur too slowly to support cellular life.
- Catalysis: Each enzyme is specific to a substrate, meaning it will only facilitate a particular reaction. This specificity ensures that metabolic pathways are finely regulated.
- Energy Production: Enzymes such as amylase and lipase help break down carbohydrates and fats, respectively, releasing energy stored in these macromolecules.
The regulation of metabolic pathways often involves allosteric interactions and feedback mechanisms, where the product of a pathway can inhibit or activate the enzymes involved. This tight regulation is essential for maintaining homeostasis within the cell.
Involvement in Signal Transduction
Signal transduction refers to the process by which cells respond to external signals through a series of molecular interactions. Proteins are central players in this process.
- Receptors: These proteins reside on the cell membrane and bind to signaling molecules, triggering a cascade of responses within the cell. For instance, insulin receptors play a critical role in regulating glucose levels.
- Second Messengers: Proteins can activate secondary messengers, like cyclic AMP, which propagate the signal within the cell, leading to various cellular responses.
This communication is crucial for coordinating activities between cells and ensuring proper responses to environmental changes. Disruptions in signal transduction pathways can lead to diseases, including cancer and diabetes.
Protein Biosynthesis
Protein biosynthesis is the process of translating genetic information into functional proteins. This is a complex and highly regulated process that is fundamental to cellular function.
- Transcription: The first step occurs in the nucleus, where DNA is transcribed into messenger RNA (mRNA). This mRNA serves as the template for protein synthesis.
- Translation: In the cytoplasm, ribosomes read the mRNA sequence and assemble amino acids in the correct order to form a polypeptide chain. tRNA molecules bring amino acids to the ribosome, ensuring that each is added according to the mRNA’s code.
Protein biosynthesis is not only about making proteins. It is also subject to regulation at multiple levels, ensuring that the cell produces proteins in response to its needs. Any disruption in this process can lead to abnormal cell function or disease.
"Proteins are often referred to as the 'workhorses' of the cell due to their diverse and critical roles in biological processes."
Protein Interactions and Complex Formation
Protein interactions and complex formation are essential aspects of protein functionality. Understanding these interactions allows researchers to comprehend how proteins operate within biological systems and how they may be manipulated for various applications. The study of protein complexes provides insights into cellular mechanisms and can lead to advancements in drug development and biotechnology.
Protein-Protein Interactions
Protein-protein interactions (PPIs) are crucial for numerous biological processes, including signaling, metabolic pathways, and structural organization of cells. These interactions can be transient or stable, depending on their nature and function.
- Types of Interactions:
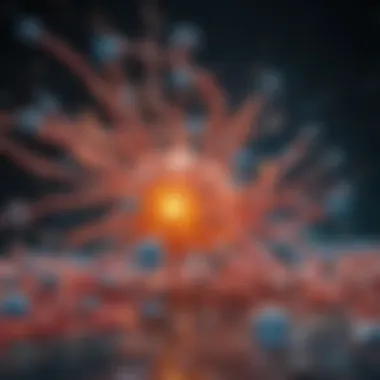
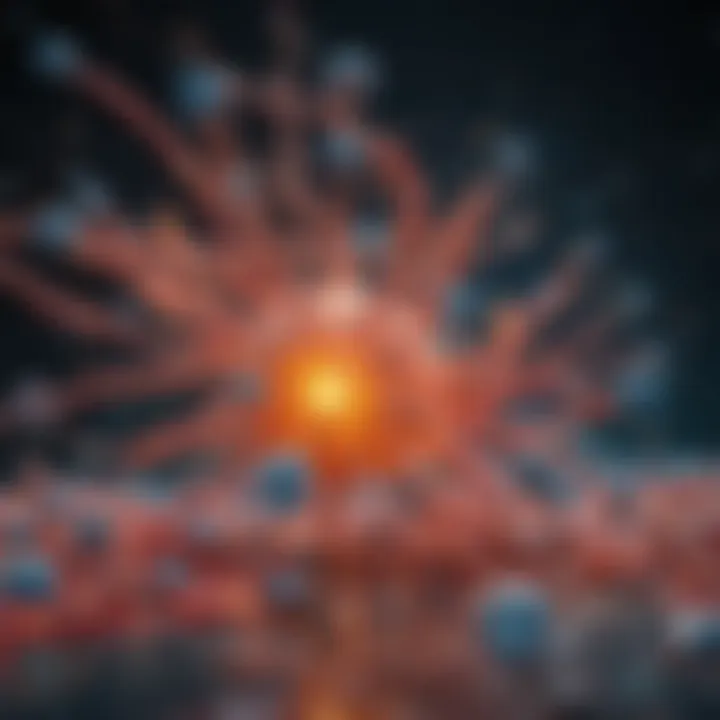
- Covalent bonds: Strong, often permanent interactions, such as disulfide bridges.
- Non-covalent interactions: Weaker, reversible interactions, including hydrogen bonds, ionic bonds, and van der Waals forces.
PPIs can form complexes that may regulate functions like enzymatic activity or signal transduction. Methods like yeast two-hybrid screening, co-immunoprecipitation, and mass spectrometry help identify and characterize these interactions, giving insight into cellular networks and pathways.
Role of Ligands in Protein Functionality
Ligands are molecules that bind to proteins, influencing their structure and function. The interaction between proteins and ligands can be critical for modulating protein activity. Ligands may be small molecules, ions, or other proteins. This binding can result in conformational changes that activate or inhibit a protein.
- Examples of Ligands:
- Hormones: These signaling molecules can bind to receptors, triggering physiological responses.
- Inhibitors: Certain drugs act as inhibitors, blocking enzyme activity or receptor function.
Understanding the role of ligands in protein functionality can assist in drug development. By knowing how a ligand interacts with a target protein, researchers can design more effective pharmaceuticals that either enhance or reduce the activity of that protein.
Impact of Post-Translational Modifications
Post-translational modifications (PTMs) are chemical modifications that occur after a protein is synthesized. These modifications can significantly influence protein functionality, stability, and localization. Common types of PTMs include phosphorylation, glycosylation, and ubiquitination.
- Phosphorylation:
- Glycosylation:
- Ubiquitination:
- This modification can activate or deactivate enzymes or receptors, playing a critical role in signal transduction.
- Adding sugar moieties can affect protein folding, stability, and recognition by other molecules.
- This modification signals proteins for degradation, thus regulating protein turnover.
As PTMs can lead to changes in protein behavior, they are significant in understanding diseases. Misregulation of PTMs could lead to issues like cancer or neurodegenerative disorders.
In summary, protein interactions and complex formation are vital for understanding cellular function. The interplay of proteins with each other, their ligands, and the impact of modifications shape the biological landscape. Research in this area is crucial for advancing scientific knowledge and therapeutic techniques.
Methods for Studying Proteins
Understanding proteins requires sophisticated methods for studying their structure and function. The choice of method depends on the specific objectives of the research and the properties of the proteins being examined. Each technique has distinct advantages and limitations, making it critical to select the appropriate one. The methods studied not only enhance our understanding of protein behaviors but also provide insights into their roles in various biological processes.
X-ray Crystallography
X-ray crystallography has been a cornerstone in the study of proteins for decades. This method allows researchers to determine the three-dimensional arrangement of atoms within a protein at atomic resolution. The significant benefit of X-ray crystallography lies in its ability to visualize protein structures in their crystallized form, revealing intricate details that are crucial for understanding protein function.
However, crystallization can be challenging. Not all proteins readily form crystals, and those that do may require extensive optimization of conditions. Despite these challenges, the data obtained from X-ray crystallography are invaluable for drug design and understanding protein-ligand interactions.
Many critical discoveries about enzyme active sites have been made using this method. For instance, the structure of the enzyme lysozyme gave insights into its catalytic mechanism, opening avenues for further research and drug development.
Nuclear Magnetic Resonance (NMR) Spectroscopy
Nuclear Magnetic Resonance (NMR) spectroscopy is another powerful technique for studying proteins. Unlike X-ray crystallography, NMR can analyze proteins in solution, offering insights into their dynamic behavior. This is important because many proteins exist in a variety of conformations in a cellular environment.
NMR provides information about protein flexibility, conformational changes, and interactions with other molecules. The technique relies on the magnetic properties of certain atomic nuclei, typically hydrogen, to yield detailed information about the protein's structure and dynamics. However, it requires relatively large amounts of protein and is limited by the size of the molecules that can be analyzed.
Despite these limitations, NMR has revealed critical information about protein folding and stability, reinforcing its importance in structural biology.
Cryo-Electron Microscopy
Cryo-electron microscopy (Cryo-EM) has emerged as a revolutionary method in protein studies, particularly for large complexes that are difficult to crystallize. This technique allows scientists to visualize proteins in their native state without the need for crystallization. By rapidly freezing proteins, Cryo-EM preserves them in a state close to their physiological conditions.
One of the most compelling advantages of Cryo-EM is its ability to analyze large macromolecular assemblies and complexes. Recently, it has been instrumental in elucidating the structures of significant cellular machinery, such as ribosomes and viruses. The resolution of Cryo-EM has improved significantly, often achieving atomic-level detail, which expands its applicability in various fields like virology and drug discovery.
Technological Applications of Proteins
The study of proteins extends beyond basic science, influencing various technological fields. Proteins are not only vital for understanding biological processes, but they also have profound technological applications. Their versatility in structure and function enables the development of innovative solutions in areas such as biotechnology, drug design, and biomaterials. The significance of these applications lies in their capacity to improve human health, advance industrial processes, and contribute to sustainable practices.
Proteins in Biotechnology
Biotechnology heavily relies on proteins to develop new tools and methods in research and industry. Recombinant DNA technology, for instance, involves modifying organisms to produce specific proteins. This can be used to generate therapeutic proteins, such as insulin or monoclonal antibodies. Understanding the properties of proteins allows researchers to fine-tune production processes, enhancing yield and efficiency. The manipulation of proteins can lead to advancements in agriculture, like the production of pest-resistant crops, thus securing food resources.
Additionally, enzymes, which are specialized proteins, play crucial roles in various biotechnological applications. They are widely used in clinical diagnostics, food processing, and bioremediation. Their specificity and efficiency in catalyzing chemical reactions make them suitable for producing biofuels and biodegradable plastics as well, contributing to environmental sustainability.
Use of Proteins in Drug Design
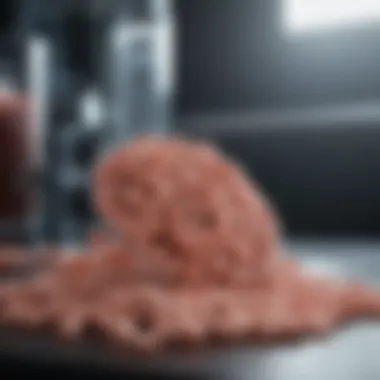
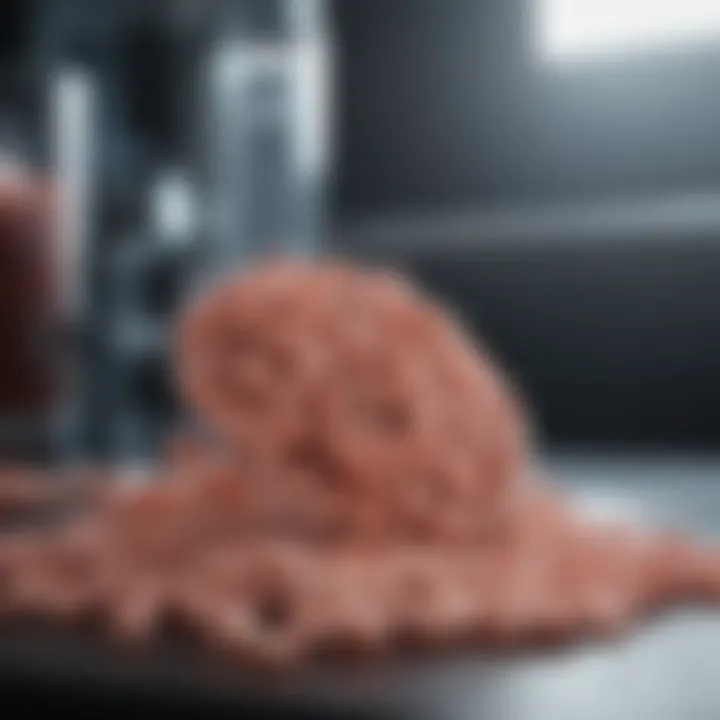
The application of proteins in drug design is another area of burgeoning importance. Proteins serve as key targets for many pharmaceuticals. Understanding protein structure and function helps pharmaceutical researchers identify potential drug binding sites. Structure-based drug design techniques utilize protein crystal structures to develop new molecules that can specifically bind to target proteins, ultimately leading to improved therapeutic efficacy.
Moreover, the emergence of biopharmaceuticals, which include proteins such as antibodies, has transformed treatment regimens for serious diseases. For example, monoclonal antibodies are designed to selectively target cancer cells, minimizing damage to healthy tissues. This specificity in action comes from a meticulous understanding of protein interactions at the molecular level.
The development of protein-based drugs is a prime example of how fundamental research leads to practical application, bridging the gap between science and therapy.
Proteins in Biomaterials
Proteins can also be utilized in the creation of biomaterials. These materials have applications in fields such as tissue engineering and regenerative medicine. For example, collagen and silk fibroin are naturally occurring proteins known for their excellent biocompatibility and mechanical properties. They provide a scaffold for cell growth, enhancing tissue repair and regeneration.
Moreover, designing biomaterials that integrate proteins can enhance drug delivery systems. By encapsulating drugs in protein carriers, researchers can achieve targeted delivery, reducing the side effects associated with traditional medications. The potential for proteins in creating biodegradable materials further exemplifies their role in sustainable practices.
In summary, the technological applications of proteins illustrate their multifaceted roles in advancing various scientific domains. As research continues to unveil the complexities of protein interactions and functions, the scope for innovative applications expands. This intersection of protein science and technology offers promising avenues for future discoveries.
Protein Misfolding and Disease
Examining protein misfolding is crucial in understanding various diseases and their underlying mechanisms. Misfolded proteins can disrupt cellular functions and ultimately lead to serious health issues. This section aims to provide insight into the nature of protein misfolding, its implications for cellular health, and insights into specific diseases that illustrate these concepts.
Understanding Protein Misfolding
Protein misfolding occurs when proteins fail to fold into their correct three-dimensional structure. This can happen due to genetic mutations, environmental factors, or errors during protein synthesis. Misfolded proteins can become dysfunctional and aggregate, leading to a range of cellular malfunctions. Understanding the mechanisms of protein misfolding offers significant benefits in the realm of biomedical research. By identifying how misfolded proteins contribute to disease, targeted interventions may be developed, potentially paving the way for effective treatments.
Impact on Cellular Function
The impact of misfolded proteins on cellular functions is profound. When proteins do not assume their intended shape, they cannot perform their specific roles, which can disrupt vital biochemical pathways. Cellular stress responses may also be activated in reaction to the accumulation of misfolded proteins. This can lead to apoptosis, the process of programmed cell death, further contributing to tissue degeneration. Studying how protein misfolding affects cellular function is crucial for developing strategies to mitigate these effects.
Examples of Misfolding Diseases
Protein misfolding is associated with a number of well-known diseases. Understanding these examples can help illuminate the broader significance of protein structure integrity in health and disease.
Alzheimer's Disease
Alzheimer's Disease is marked by the accumulation of amyloid-beta plaques and tau protein tangles. These misfolded proteins interfere with neuronal communication. The progressive nature of Alzheimer's makes it a common choice for study in protein misfolding research. One key characteristic is the role of amyloid plaques in disrupting brain function. This disease serves as a prominent example of how misfolded proteins can lead to cognitive decline and has considerable implications for aging populations.
Parkinson's Disease
Parkinson's Disease features the aggregation of alpha-synuclein protein, which forms Lewy bodies within neurons. This condition particularly affects movement, leading to tremors and rigidity. Highlighting Parkinson's Disease is beneficial because it showcases how specific misfolding events can result in debilitating motor dysfunction. Furthermore, studying this disease provides insights into neurodegeneration and strategies to potentially alter the disease's trajectory.
Cystic Fibrosis
In Cystic Fibrosis, a common mutation causes the CFTR protein to misfold, preventing it from reaching its destination in the cell membrane. This misfolding leads to severe respiratory and digestive issues. Understanding Cystic Fibrosis is crucial because it addresses a genetic basis for protein misfolding and its broad effects on cell physiology. This disease emphasizes the need for therapies focused on correcting protein folding as a treatment strategy.
"The study of protein misfolding not only illuminates disease mechanisms but also highlights potential therapeutic approaches to combat these maladies."
Through the exploration of these diseases, we see how critical the proper folding and function of proteins are to overall health. Addressing misfolding provides a pathway toward innovative treatments and better patient outcomes.
Future Directions in Protein Research
The field of protein research is poised at a pivotal moment, where advancements in technology and scientific understanding can redefine how we approach biological systems. Future directions in this realm are not just exciting but vital for unraveling the complexities of life at a molecular level. This section addresses specific elements such as emerging techniques, the potential for synthetic biology, and the challenges that the research community must navigate. Understanding these aspects enhances the relevance of protein studies across multiple scientific disciplines, including medicine, biotechnology, and environmental science.
Emerging Techniques in Protein Analysis
The array of techniques available for protein analysis continues to expand, promising more precise and insightful results. Among the most notable emerging methods are:
- Mass Spectrometry: This technique enables high-throughput analysis, identifying proteins based on their mass-to-charge ratios.
- Next-Generation Sequencing: As a powerful tool, it allows researchers to investigate protein coding genes comprehensively.
- Single-Molecule Techniques: Innovations like optical tweezers and fluorescence correlation spectroscopy can provide detailed insight into protein behavior in real-time.
These methods offer researchers a deeper understanding of protein dynamics, interactions, and functions. They can also enhance drug discovery processes and contribute to personalized medicine approaches, making results more tailored to individual patients.
Potential for Synthetic Biology
Synthetic biology represents a promising frontier in protein research. By leveraging the principles of engineering, synthetic biology seeks to design and construct new biological parts and systems. Some potentials include:
- Custom Protein Creation: Researchers can design proteins for specific functions, which can have applications in therapeutics and diagnostics.
- Biomolecular Machines: With advanced genetic circuits, creating protein-based machines could lead to novel therapeutic strategies and drug delivery systems.
- Biofuels and Bioremediation: Engineered proteins can enhance the efficiency of microbial processes, contributing to sustainable energy solutions and environmental cleanup efforts.
The influence of synthetic biology in protein research expands the horizons of what is possible. However, ethical considerations and safety protocols must be prominent in these discussions to ensure responsible application.
Challenges Ahead in Protein Research
Despite the advancements, several challenges persist in the domain of protein research. These include:
- Complexity of Protein Structures: Understanding the intricacies of protein folding and stability remains a significant challenge, especially in the context of diseases.
- Data Interpretation: The influx of data from advanced techniques requires careful analysis and interpretation, which often demands sophisticated bioinformatics tools.
- Funding and Accessibility: Securing funding for cutting-edge research can be difficult. Furthermore, there is a need for open access to databases and publications to encourage collaborative efforts.
Addressing these challenges is crucial for the future of protein research. Collaborative work among academia, industry, and regulatory bodies will play an essential role in overcoming these obstacles.
"As we forge ahead, the intersection of technology and biology will be key in unlocking the full potential of proteins in scientific research."