RNA and Protein Synthesis: A Deep Dive
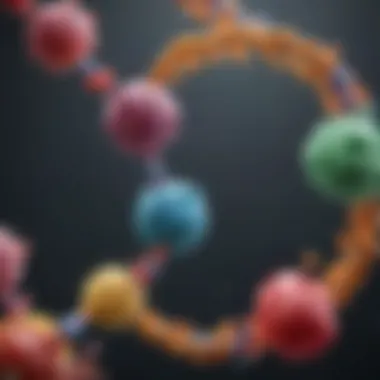
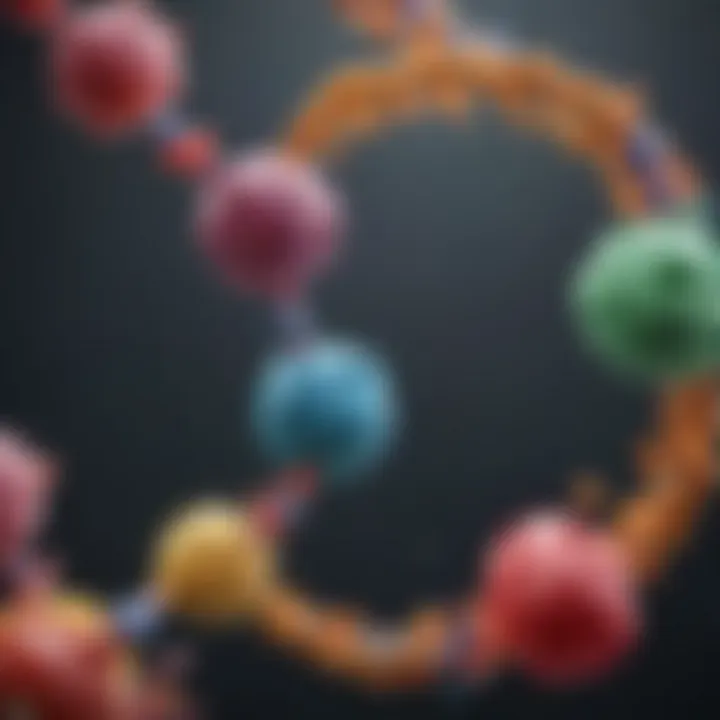
Intro
In the realm of cellular biology, the processes of RNA and protein synthesis stand as fundamental pillars, intricately woven into the fabric of life itself. These processes are not just mere biochemical reactions; they are the very language through which genetic information is translated into functional organisms. The central dogma of molecular biology serves as a guiding framework, illustrating how DNA is transcribed into RNA and subsequently translated into proteins. This article takes a closer look at each of these critical steps, unpacking the minutiae of transcription and translation while also considering regulatory mechanisms and their implications in health and disease.
The journey begins with an examination of various types of RNA, all of which play distinct roles within the cell. Messenger RNA (mRNA), ribosomal RNA (rRNA), and transfer RNA (tRNA) each serve as crucial players in the protein synthesis saga. As we navigate through these biomolecules and their functions, the focus narrows to the methods cells employ to orchestrate the remarkable feat of transforming genetic blueprints into tangible life forms.
Furthermore, we delve into contemporary research trends that illustrate how understanding RNA and protein synthesis intersects with areas such as genetic disorders, cancer research, and even evolutionary biology. By grasping the complexities of these processes, one can appreciate how they influence not just individual health but the very evolution of species.
Methodologies
Description of Research Techniques
Unraveling the intricate processes of RNA and protein synthesis involves several sophisticated research techniques. One key method is polymerase chain reaction (PCR), which allows scientists to amplify small segments of RNA to examine them in greater detail. This technique has revolutionized the ability to study genetic material, providing a clearer view into the dynamics of transcription and translation.
Another significant approach is Northern blotting, which helps researchers assess the size, abundance, and integrity of RNA molecules. By isolating RNA samples from cells, scientists can visualize the different RNA species present, further informing our understanding of how these molecules function in various biological contexts.
Western blotting is often employed in protein analysis, enabling the detection and quantification of specific proteins within a sample. By combining these methodologies, researchers draw connections between RNA expression patterns and protein production, fostering insights into cellular responses to a variety of stimuli.
Tools and Technologies Used
A robust array of tools empowers researchers in their quest to decode RNA and protein synthesis. Next-generation sequencing (NGS) stands out as a game changer, offering unprecedented speed and accuracy in sequencing RNA molecules. This technology has permitted researchers to analyze transcriptomes, presenting a more comprehensive overview of gene expression under various conditions.
For real-time observation of protein synthesis, fluorescence microscopy and live-cell imaging techniques are invaluable. These cutting-edge tools enable the visualization of proteins in action within living cells, shedding light on dynamic processes like translation and cellular interactions that were previously difficult to capture.
Moreover, CRISPR-Cas9 gene-editing technology has opened new avenues for understanding the impacts of specific genes on RNA and protein synthesis. By creating targeted mutations, researchers can observe resulting changes in cellular behavior, thus revealing deeper insights into the underlying mechanisms at play.
Key Insight: The combination of advanced sequencing methods, imaging technologies, and gene-editing tools continues to refine our comprehension of RNA and protein synthesis, driving forward biological research at an unprecedented pace.
Discussion
Comparison with Previous Research
When examining RNA and protein synthesis, it is crucial to analyze how these insights compare with earlier findings. Historically, studies focused primarily on isolated aspects of these processes, often neglecting the intricate relationships between RNA types and their regulatory roles. Modern approaches emphasize a more integrated view, studying how various RNA species interact and collaborate in protein creation, thus painting a more complex and accurate picture.
Theoretical Implications
The implications of RNA and protein synthesis extend beyond the laboratory. Theoretical frameworks based on these processes help to explain phenomena in health and disease. For example, mutations in RNA processing mechanisms have been linked to various genetic disorders, providing concrete evidence that our understanding of RNA is crucial for medical advancements. Conversely, the exploration of protein synthesis pathways has implications for drug development, particularly in targeting protein interactions that drive diseases like cancer.
Overview of Molecular Biology
Definition of Molecular Biology
Molecular biology can be defined as the branch of science that focuses on the structure and function of the molecules essential for life. This involves studying nucleic acids (like DNA and RNA) and proteins, dissecting how they interact with each other to sustain life. The study is pivotal because it offers insights into how genetic information is stored, replicated, and expressed in living organisms. Thus, knowledge gained here is not only fundamental for academic growth but also for practical applications, such as genetic engineering and pharmaceuticals.
Importance of RNA and Proteins
RNA and proteins play indispensable roles in the fabric of life. RNA, which serves as the messenger and executor of genetic instructions, is vital for the translation of DNA's blueprint into functional proteins. Proteins, in turn, act as the workhorses of the cell, involved in virtually every cellular function from catalyzing chemical reactions to providing structural support. Without these two components, life as we know it would not exist. Their study is increasingly important as we seek to understand development, health, and disease.
Central Dogma of Molecular Biology
The concept of the Central Dogma is a cornerstone of molecular biology, articulating the flow of genetic information within a biological system. This principle is underscored by three key processes: DNA replication, transcription to RNA, and translation to protein.
DNA Replication
DNA replication is a fundamental process that ensures genetic material is copied accurately so that new cells receive the same genetic information as their predecessors. This mechanism is characterized by its semi-conservative nature; each new double helix consists of one old strand and one new strand. It's regarded as a critical choice for this discussion due to its role in maintaining genetic stability and integrity, which are paramount for the proper functioning of living organisms. One unique feature of DNA replication is its error-checking capabilities, which significantly reduces mutations, though it may not always catch every slip-up, leading to genetic diversity.
Transcription to RNA
The transcription process converts DNA into RNA and is a vital step in gene expression. By producing messenger RNA (mRNA), this step allows for the transfer of genetic information from the secure sanctuary of DNA in the nucleus out to the cytoplasm, where proteins are synthesized. The key characteristic of transcription is its regulation; not all genes are transcribed simultaneously or equally, thus allowing for adaptability within cells. A noteworthy advantage of this process is its versatility, allowing cells to respond quickly to environmental changes by altering gene expression patterns. However, selectively transcribing genes can also have disadvantages if certain critical genes are not expressed when needed.
Translation to Protein
Translation is the final phase of gene expression, where the information carried by mRNA is converted into protein. This process takes place in ribosomes and involves various types of RNA, including transfer RNA (tRNA) that delivers amino acids. The defining feature of translation is its specificity; each codon on the mRNA corresponds to a particular amino acid, ensuring that proteins are built correctly. This method of protein production is beneficial for sustaining cellular functions, as proteins execute diverse roles within the cell. Nonetheless, errors in translation can lead to malfunctioning proteins, which might contribute to diseases.
"The simplicity of the genetic code carries profound implications for both understanding life and manipulating it through technologies like CRISPR."
Types of RNA
Messenger RNA (mRNA)
Messenger RNA (mRNA) is often dubbed the middleman in the flow of genetic information. It’s like a shuttle bus that transports messages from the DNA in the nucleus of a cell to the ribosomes where proteins are synthesized. When a gene is expressed, an RNA polymerase enzyme synthesizes mRNA from the DNA template in a process known as transcription. This mRNA strand carries the specific instructions needed to build proteins, signifying the link between the genetic code and the functional proteins. Its sequence of nucleotides corresponds directly to the amino acids that will be joined together to form proteins.
Transfer RNA (tRNA)
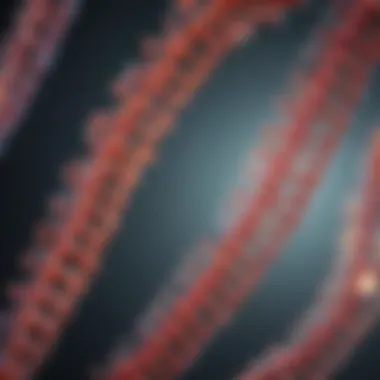
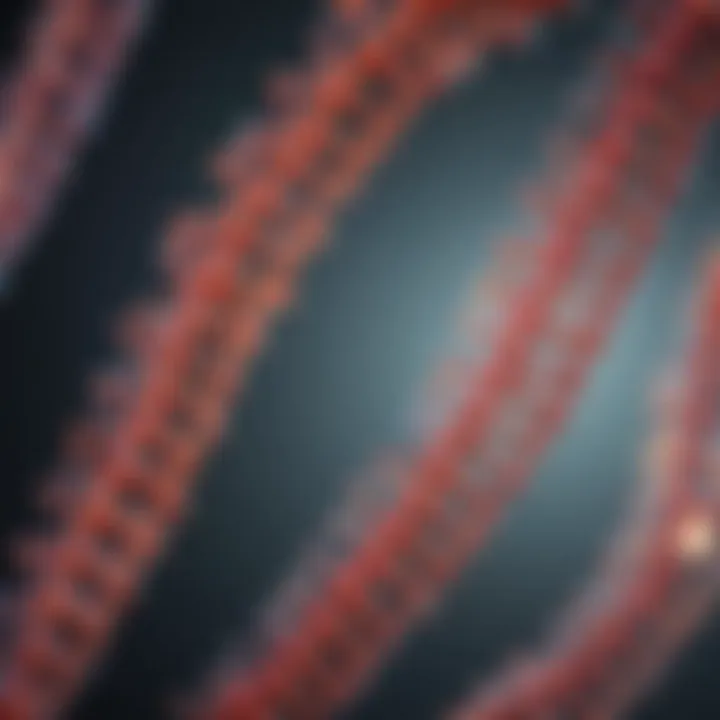
Transfer RNA (tRNA) is the workhorse that brings the amino acids to the ribosome during protein synthesis. Think of tRNA as the delivery person who picks up the right "ingredients" and delivers them to the "cooking" site—the ribosome. Each tRNA has an anticodon, a three-nucleotide sequence that pairs with the corresponding codon on the mRNA, ensuring that the correct amino acid is added in the proper sequence. This specificity is crucial; if the wrong amino acid is integrated, it can lead to faulty proteins, which may result in various cellular dysfunctions.
Ribosomal RNA (rRNA)
Ribosomal RNA (rRNA) is a structural component of ribosomes, the cellular machinery that orchestrates protein synthesis. rRNA is not merely a passive player; it also possesses enzymatic properties, facilitating the chemical reactions that form peptide bonds between amino acids. In a sense, rRNA is like the glue that holds everything together during the translation process, playing a pivotal role in ensuring that proteins are produced efficiently and accurately.
Non-Coding RNAs
While the primary focus often rests on coding RNAs like mRNA, non-coding RNAs (ncRNAs) are essential players in the regulation and expression of genes. These molecules, which do not translate into proteins themselves, participate in numerous cellular processes, including gene regulation, chromatin organization, and even as precursors to regulatory RNA forms.
Functions of Non-Coding RNAs
The functions of non-coding RNAs are diverse and significant. They play roles in regulating gene expression at several levels. For instance, microRNAs (miRNAs) can bind to mRNAs and inhibit their translation, while long non-coding RNAs (lncRNAs) can modify chromatin structure to enhance or repress certain genes.
Non-coding RNAs shape not just gene activity but also the trajectory of cell development and differentiation.
This unique feature of non-coding RNAs makes them a valuable area of study, particularly in understanding diseases like cancer where gene expression may be dysregulated.
Types of Non-Coding RNAs
Non-coding RNAs can be categorized into several types, each with its specific functions and characteristics. Examples include:
- MicroRNAs (miRNAs): Short, single-stranded RNA molecules that regulate gene expression by degrading mRNA or inhibiting translation.
- Long Non-Coding RNAs (lncRNAs): Longer RNA sequences that can regulate gene expression at various levels, impacting both transcription and post-transcription processes.
- Small Nuclear RNAs (snRNAs): Involved in the processing of pre-messenger RNAs in the spliceosome.
The distinct diversity of non-coding RNAs demonstrates their crucial importance in cellular biology. While they do not produce proteins directly, their regulatory roles are fundamental in determining how and when genes are expressed, underscoring their significance in molecular pathways.
Transcription Process
Transcription is the first major step in the complex journey of gene expression, translating genetic information from DNA into RNA. This crucial process forms the foundation for everything that happens next—namely, translation, which subsequently synthesizes proteins. Understanding transcription highlights its importance in both cellular functionality and broader biological systems, and serves as a gateway to the intricate mechanics of life.
Initiation
Initiation marks the beginning of transcription, setting the stage for RNA synthesis. The recognition of specific DNA sequences that signify where transcription should start dominates this phase. This process is not merely an arbitrary selection; it is pivotal in defining how and when genes are expressed.
Promoter Recognition
Promoter recognition is the initial pinpoint in the transcription timeline. Here, specialized sequences in the DNA, known as promoters, play a crucial role. These regions are located upstream of the gene and essentially act as anchors for the transcription machinery to attach. One key characteristic of promoter recognition is its specificity—specific sequences are recognized by proteins called transcription factors. These elements are beneficial because they ensure that the correct genes are activated at the right time, in turn influencing cellular behavior.
A unique feature of promoter recognition is its variability across different organisms and cell types. Some promoters require the presence of additional elements to facilitate binding—a hallmark of more complex regulatory mechanisms. This can be advantageous, allowing cells to finely tune gene expression in response to internal or external stimuli, though it can hinder rapid responses due to the need for multiple factors.
Transcription Factors
Transcription factors are proteins that bind to specific DNA sequences, playing a critical role in modulating transcription processes. Their contribution to gene activation, or sometimes repression, makes them a focal point in understanding the regulation of the transcription process. What stands out about transcription factors is their ability to act as conductors in the symphony of gene expression, orchestrating when and how genes are expressed based on signals from the environment or developmental cues.
A key characteristic of transcription factors is their modular structure, composed of distinct domains that can bind to DNA or interact with other proteins. This modularity provides flexibility, enabling them to form complexes with other proteins and respond to various signals efficiently. However, these factors can also have disadvantages; deregulation can lead to inappropriate gene expression, contributing to diseases like cancer. Their capacity for both enhancement and repression makes them essential players in the transcription initiation.
Elongation
Following initiation, elongation begins as RNA polymerase moves along the DNA strand, synthesizing an RNA strand by adding nucleotides complementary to the template DNA. This stage ensures that a complete and accurate RNA copy is generated, crucial for downstream processes. The elongation phase aids in integrating various splicing mechanisms that prepare the mRNA for its eventual role in protein synthesis.
Termination
Termination is the concluding step in transcription where the RNA polymerase encounters termination signals in the DNA sequence. This process is as essential as initiation and elongation, as it ensures that the transcription machinery stops at the right place. An accurate termination enables the synthesis of RNA transcripts that are aptly sized for subsequent processes and diminishes the chance of errors. Proper termination guarantees that the RNA product is ready for the next phase in the gene expression continuum.
Translation Mechanism
Translation is the crucial next step in the protein synthesis process, following transcription. This mechanism converts the genetic information coded in messenger RNA (mRNA) into a functional protein, which is essential for the myriad of functions carried out within living organisms. Understanding this process not only deepens our knowledge of cellular biology but also aids in innovations in biotechnology and medicine. Specifically, this section focuses on the components of translation, the roles each plays, and how they interplay to produce proteins that are vital for life.
Ribosomes in Translation
Ribosomes serve as the protein factories of the cell. These complex structures are made up of ribosomal RNA (rRNA) and proteins that come together in two subunits – the large and the small. The ribosome reads the sequence of mRNA codons and translates them into amino acids through a process that involves decoding the genetic instructions embedded in RNA.
The ribosome operates like a conductor leading an orchestra, where each player must perform in harmony.
- Structure: The small subunit binds to the mRNA strand first. Once anchored, the large subunit attaches to form a complete ribosome ready for translation.
- Function: The ribosome facilitates the binding of tRNA, allowing for the transfer of the appropriate amino acids. The precision and efficiency of ribosomes make them indispensable in biological processes.
Role of tRNA
Transfer RNA (tRNA) is an essential player in translation. Each tRNA molecule has a specific amino acid attached to it, and it recognizes corresponding codons on the mRNA sequence via its anticodon. In essence, tRNA is the connector between the coded genetic information and the physical proteins made from that code.
- Delivery System: Think of tRNA as a delivery truck, transporting the right amino acid to the ribosome.
- Accuracy: The accuracy of this process is critical; if the wrong amino acid is delivered, it can have huge implications for protein function.
Peptide Bond Formation
Once the appropriate tRNA brings an amino acid to the ribosome, peptide bonds are formed between amino acids. This process occurs in the active site of the ribosome, specifically in the large subunit. Peptide bonds are covalent linkages that occur through a dehydration reaction, where a water molecule is lost as the amino acids come together.
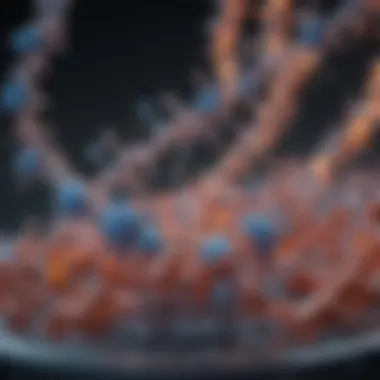
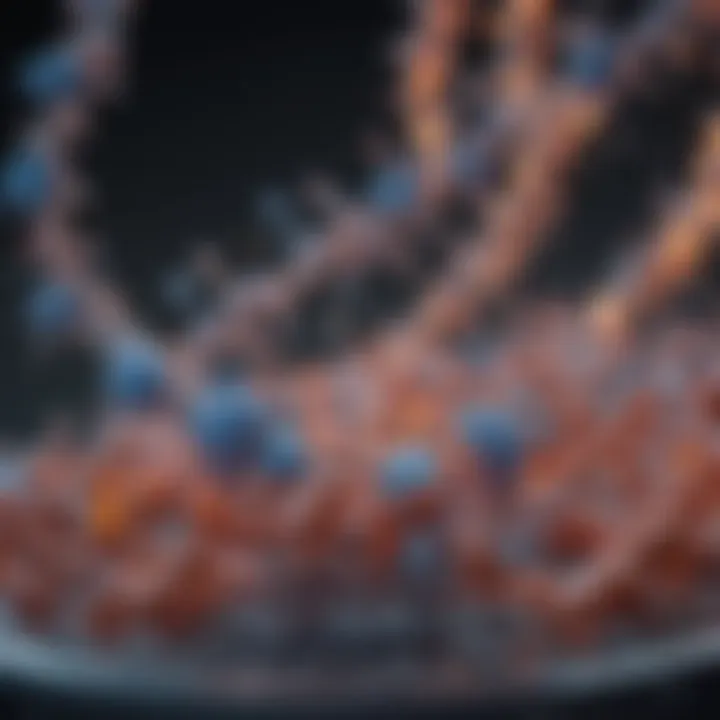
- Mechanism: The ribosome catalyzes this reaction, facilitating the formation of a growing polypeptide chain.
- Importance: Each peptide bond represents a moment of molecular significance, ultimately determining the protein's structure and function.
Translation Termination
Translation does not go on indefinitely; it has a clear endpoint known as termination. This occurs when a stop codon on the mRNA strand is reached by the ribosome.
- Signal Recognition: Stop codons do not code for any amino acid. Instead, they serve as termination signals, prompting the release factors to intervene.
- Release of Polypeptide: Upon reaching a stop codon, the newly formed polypeptide chain is released, and the ribosomal subunits disassemble, preparing them for the next round of translation.
Understanding translation termination is fundamental, as it signifies the completion of a protein synthesis cycle and allows cells to regulate their protein production effectively.
In sum, the translation mechanism encapsulates a series of well-orchestrated steps that are crucial for cellular function. With each tRNA bringing amino acids to the ribosome, peptide bonds forming to build proteins, and ribosomes ensuring fidelity, this intricate dance of molecular choreography synthesizes proteins that carry out vital roles in almost every aspect of life.
Regulatory Mechanisms
The role of regulatory mechanisms in RNA and protein synthesis cannot be overstated. These processes are essential for fine-tuning gene expression, allowing cells to adapt and respond to their environment. By controlling these molecular interactions, cells can optimize the production of proteins where and when they are needed. This dynamic regulatory framework is vital for maintaining cellular homeostasis and overall organism health.
Gene Regulation
Transcriptional Regulation
Transcriptional regulation is the first step in controlling gene expression. It refers to the processes that initiate and maintain the transcription of specific genes into messenger RNA. This regulation is crucial because it sets the stage for how much protein will ultimately be synthesized.
One of the key characteristics of transcriptional regulation is its ability to respond to various signals, such as hormones or environmental factors. For instance, when certain stressors are present, transcription factors can enable or inhibit the transcription of specific genes to help the cell navigate through the situation. This responsiveness makes transcriptional regulation a popular focus for research.
A unique feature of transcriptional regulation is the use of promoter regions and enhancers. Promoters are sequences located at the start of a gene that are recognized by RNA polymerase, the enzyme responsible for transcribing RNA. Enhancers, on the other hand, can be situated far away from the gene they regulate but still influence its expression positively or negatively.
While the advantages of transcriptional regulation are clear—such as swift responses to environmental changes—there are some disadvantages. Misregulation at this stage can lead to diseases, including cancer, where improper gene expression can contribute to uncontrolled cell growth.
Post-Transcriptional Regulation
Post-transcriptional regulation involves modifications after the transcription phase, impacting the mRNA before it is translated into a protein. This regulation is critical because it adds another layer of control in gene expression. By fine-tuning this process, cells can decide which mRNAs are translated and when, effectively modulating protein levels without altering transcription rates.
The key characteristic of post-transcriptional regulation lies in its ability to manipulate mRNA stability and translation efficiency. For instance, certain molecules can bind to mRNA and either promote degradation or enhance its translation, which is especially vital during cellular stress or development.
One unique aspect of post-transcriptional regulation is the role of small non-coding RNAs, such as microRNAs, which can inhibit translation by binding to complementary sequences on target mRNAs. This allows for a sophisticated control system to adjust protein production as needed.
Advantages of post-transcriptional regulation include its speed and efficiency in responding to immediate cellular needs. However, it can also lead to complexities. For instance, misregulation can result in the production of faulty proteins or insufficient protein levels, both of which can have significant implications for cellular function and health.
Post-Translational Modifications
Post-translational modifications (PTMs) refer to the chemical modifications that proteins undergo after their synthesis. These modifications can include phosphorylation, glycosylation, ubiquitination, and more. Each type of PTM carries its own implications for the protein’s behavior and function.
Because proteins may need to be activated or localized to different cellular compartments, PTMs offer essential flexibility. For instance, phosphorylation can modulate enzyme activity, while glycosylation may be crucial for protein stability or cell-cell recognition. This variety ensures that proteins can perform their functions in diverse contexts, adapting to changes in cellular demands.
However, PTMs can also complicate our understanding of proteins. Different modifications on the same protein can yield various functional outcomes—this could be a double-edged sword.
"In the world of molecular biology, regulation is not just a luxury; it's a necessity for survival, allowing organisms to respond to a vast array of stimuli in real time."
Emerging Research and Applications
The field of RNA and protein synthesis is not stagnant; it evolves perpetually, driven by groundbreaking discoveries and technological advancements. As researchers delve deeper into the genetic blueprints of organisms, emerging research is reshaping our understanding and expanding the applications of RNA and proteins in health care, biotechnology, and evolutionary biology. This section navigates the significant developments in three pivotal areas: CRISPR and gene editing, RNA-based therapeutics, and protein engineering. Each of these avenues offers transformative potential that could redefine medicine and our approach to biological problems.
CRISPR and Gene Editing
One of the stars in the contemporary scientific arena is undoubtedly CRISPR, short for Clustered Regularly Interspaced Short Palindromic Repeats. This innovative gene-editing technology has revolutionized how we consider genetic manipulation. By enabling precise alterations to DNA, CRISPR opens doors to correcting genetic disorders at their roots. Its appeal lies in the simplicity and efficiency it offers when compared to earlier gene-editing methods.
What’s particularly exciting is the adaptability of CRISPR. Scientists have expanded its applications beyond basic genetic engineering to include therapy for diseases like sickle cell anemia and certain forms of cancer. Imagine a world where genetic diseases might be directly edited out—this possibility isn't just a far-fetched dream anymore. Researchers are honing the CRISPR technique to ensure its accuracy and minimize off-target effects.
"The day is not far when genetic diseases may be treated like software bugs—just edit them out!"
As they tread carefully, considering ethical implications, practical applications remain at the forefront of discussions. The potential for CRISPR applications in agriculture to produce resilient crops is also something that cannot be overlooked. Thus, CRISPR stands at a fascinating intersection of technology, ethics, and science, pushing us to rethink what we know about genetic engineering.
RNA-Based Therapeutics
The role of RNA is not limited to its function as a messenger—it has stepped into the spotlight with the rise of RNA-based therapeutics. These are particularly pivotal in enhancing medical treatments, especially with regards to viral infections and genetic disorders. One such advancement is the use of messenger RNA (mRNA) vaccines, famously brought to prominence with the COVID-19 pandemic. These vaccines have showcased how mRNA can instruct cells to produce antigens, triggering an immune response without using live pathogens.
Besides vaccines, researchers explore RNA's potential in treating diseases at the genetic level. siRNA and antisense oligonucleotides are two examples paving the way for therapies targeting RNA transcripts, leading to the silencing of disease-causing genes. This specificity is a game-changer in treating diseases that traditional methods struggle to address.
Yet, questions remain on delivery mechanisms and the stability of these therapies in the body. Ongoing research is tackling these challenges, pushing the boundaries of what RNA can accomplish in the therapeutic realm. Innovators are also looking at ways to improve the reliability and efficacy of these RNA-based tools.
Protein Engineering
Protein engineering is another frontier where RNA and protein synthesis find exciting applications. By manipulating the genetic codes that dictate protein formation, scientists can create novel proteins with enhanced functions or reduced side effects. This discipline merges chemistry, biology, and engineering, and it's filled with possibilities.
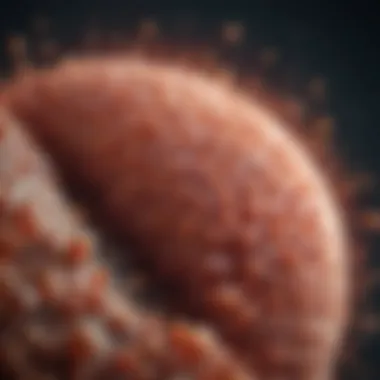
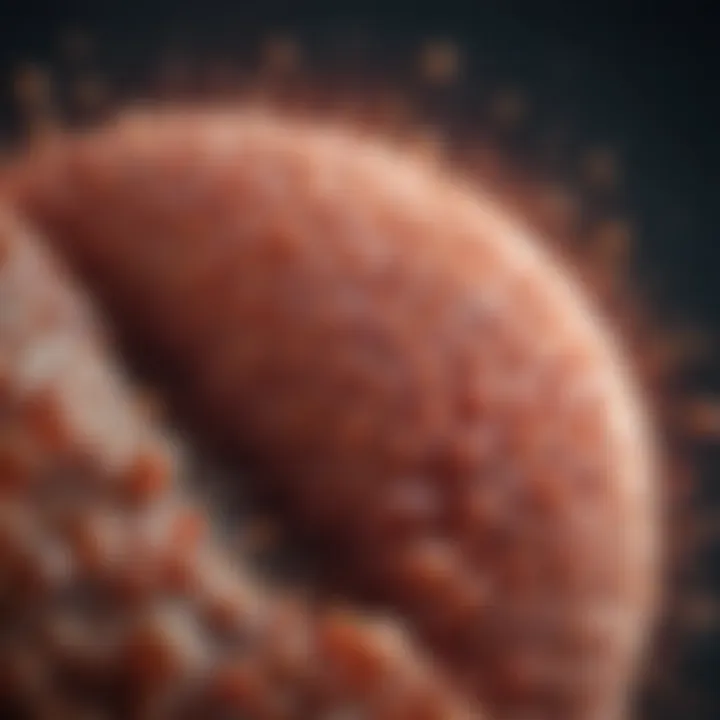
From industrial applications, like developing enzymes for greener manufacturing processes, to designing proteins that can act as drugs or even catalysts in reactions, the potential is vast. One notable example is designed antibodies that can precisely target specific cells or pathogens without affecting healthy ones. This targeted approach is invaluable in cancer treatment and could lead to significant advancements in personalized medicine.
Protein engineering also extends to biotechnology, where synthetic proteins could be used in biofuels, waste management, and even food production.
In summary, the landscape of RNA and protein synthesis is rapidly evolving. Through advances in CRISPR technology, innovative RNA-based treatments, and the burgeoning field of protein engineering, significant strides are being made that hold promise for both therapeutic and practical applications. The implications of these developments not only influence healthcare but echo throughout biotechnology and beyond, paving the way for discoveries that can reshape our understanding and interaction with biological systems.
Implications in Health and Disease
Understanding the implications of RNA and protein synthesis is like reading the blueprint of life itself. These processes not only drive the basic cellular functions but also lay the groundwork for understanding a variety of health conditions. In health and disease, the proper functioning of RNA and proteins is fundamental. Any misstep in these processes can lead to significant consequences, illustrating their importance in modern medicine.
Genetic Disorders
Genetic disorders arise from anomalies in the DNA sequence, which directly affect RNA and protein synthesis. When mutations occur within genes, they often disrupt the transcription process, resulting in altered mRNA that codes for dysfunctional proteins. One example is Cystic Fibrosis, caused by mutations in the CFTR gene, leading to faulty protein production that impairs chloride transport in epithelial cells. This highlights how a single genetic defect can cascade into broader health issues, affecting numerous bodily systems.
The role of RNA in such conditions is growing in importance. For instance, research into RNA interference (RNAi) shows promise for targeting and silencing defective genes, offering potential treatments for various genetic disorders. This targeted approach opens new avenues for therapeutic development, pushing the boundaries of traditional gene therapy.
Cancer Biology
Cancer biology intricately links to RNA and protein synthesis, as cancerous cells exhibit uncontrolled growth driven by altered gene expression. Mutations can activate oncogenes – genes that promote cell division – or deactivate tumor suppressor genes. Consequently, the balance of RNA transcription processes becomes disrupted, allowing for unchecked protein synthesis that leads to tumor development.
Interestingly, many cancer treatments are being developed that target these specific RNA and protein pathways. For instance, small interfering RNAs (siRNAs) are being harnessed to silence oncogenes selectively. Additionally, understanding the post-translational modifications of proteins in cancer can reveal potential therapeutic targets, making the connection between RNA synthesis and cancer treatment an area of extensive research.
Infectious Diseases
Infectious diseases also underline the significance of RNA and protein synthesis. Pathogens, including viruses, often hijack the host's cellular machinery to replicate their own RNA and proteins, thus propagating the infection. The influenza virus, for example, utilizes the host's RNA polymerase to replicate its RNA, fundamentally compromising the host's immune response.
On the therapeutic side, advancements in antiviral drugs have been largely focused on disrupting these processes. Medications that inhibit viral RNA synthesis or interfere with protein translation have become essential tools in combating viral infections. The rapid development of mRNA vaccines for diseases like COVID-19 showcases how understanding these mechanisms can lead to innovative solutions in public health.
The interplay between RNA, protein synthesis, and disease states reveals the intricate web of molecular interactions that underpin life. Each breakthrough in understanding these processes paves the way for novel applications in treatment and management of diseases.
Evolutionary Perspectives
The study of RNA and protein synthesis can’t be fully appreciated without understanding its evolutionary backdrop. This perspective sheds light on how these biological processes have shaped life over billions of years. By exploring the evolutionary lens, we gain valuable insights into why certain mechanisms function as they do today and how various organisms have adapted their molecular biology over time.
Origin of RNA
The origin of RNA is a captivating topic in molecular evolution. It is widely believed that RNA preceded DNA as the primary genetic material in early life forms. This idea is often supported by the RNA world hypothesis, which posits that self-replicating RNA molecules could have given rise to today's complex life.
- RNA possesses both the capacity to store genetic information and to catalyze biochemical reactions. This dual function makes it a prime candidate for early life processes.
- The transition from RNA to DNA represents a significant evolutionary event. DNA is more chemically stable, which allowed for more extensive genetic information preservation.
However, the journey from simple RNA molecules to the elaborate systems seen in modern cells involved countless evolutionary steps. Understanding this transition is crucial because it reflects the adaptability of life. RNA’s ability to evolve, replicate, and engage in catalysis likely laid the groundwork for the diverse range of organisms we see today.
Evolution of Genetic Codes
Diving deeper, the evolution of genetic codes reveals how molecular biology has been fine-tuned through natural selection. From the universal code that governs most forms of life to more specialized codes in various organisms, this evolution speaks volumes about adaptability.
- Universal Code: The fact that nearly all living organisms employ the same genetic code hints at a shared ancestor. This efficiency suggests a strong selection pressure for this system.
- Variations: Yet, in different domains of life, variations exist. For instance, certain codons in mitochondria differ from standard codes. This hints at the unique evolutionary paths certain organisms have taken.
Thus, these variations tell stories of adaptations to specific environments. Natural selection has shaped these codes, enhancing the fitness of organisms based on their surroundings.
Natural Selection of Proteins
When we look at proteins, we observe the outcome of countless molecular trials filtered through natural selection. Proteins are the workhorses of the cell, performing a plethora of functions from catalyzing reactions to providing structural support. Understanding how proteins evolved provides insight into how complex cellular functions emerged.
- Each protein structure is a result of the amino acid sequence determined by the underlying genetic information. Variations in this sequence arise due to mutations, which, over time, are subject to the whims of natural selection.
- Proteins that confer a survival advantage get retained within a species, while those that do not tend to fade out. For example, enzymes that can efficiently process available resources lead to better energy uptake and faster growth.
"Natural selection doesn't just shape the present; it carves out the future of life, defining which traits persist and which fade into the annals of extinction."
The evolution of proteins illustrates a dynamic interplay between genetic variability and environmental challenges. As conditions change, the proteins that function most effectively provide a selective edge, showcasing evolution at work.
In essence, the evolutionary perspectives on RNA and protein synthesis help us understand the interplay of genetics, environment, and adaptation, crafting the tapestry of life as we know it today.
Ending and Future Directions
In the vast arena of molecular biology, the conclusion and future directions surrounding RNA and protein synthesis stand testament to the enduring relevance of these processes. Understanding these elements (they're no small potatoes!) is crucial for multiple reasons. Not only do they shed light on fundamental biological mechanisms, but they also provide a roadmap for potential advancements in genetic research and therapeutic applications. As we draw the curtains on our exploration, it's clear that this content is more than just textbook knowledge—it's a gateway into innovative research and therapeutic breakthroughs that have profound implications for health, disease management, and technology.
Summary of Key Findings
In detailing the journey from DNA to functional proteins, several key findings emerge:
- Central Dogma: The journey of information transfer—from DNA to RNA and then to proteins—underscores the complexity and precision of genetic expression.
- Types of RNA: Each RNA type plays distinct roles, with mRNA acting as a messenger, tRNA as a translator, and rRNA as a structural component in ribosomes, proving vital in the translation process.
- Regulatory Mechanisms: Gene regulation emerges as a pivotal player in cellular function, governing when, where, and how genes are expressed, which is particularly significant within the context of disease and adaptation.
- Emerging Technologies: Innovations like CRISPR have opened doors to gene editing, bringing about new methodologies to address genetic disorders and enable precise modifications.
These points reflect the critical essence of RNA and protein synthesis, not just as mechanistic functions but as fundamental processes driving life.
Potential Research Avenues
Looking ahead, potential research avenues are as varied as they are promising:
- RNA-Based Therapeutics: The application of RNA in treatments, such as mRNA vaccines against viral infections, represents a paradigm shift in how we address diseases. Further research into RNA nanotechnology could provide solutions for targeted drug delivery.
- Protein Engineering: as the demand for tailored proteins rises, understanding the underpinning mechanisms for protein folding and structure can lead to breakthroughs in industrial and therapeutic protein production.
- Gene Regulatory Networks: Delving deeper into how genes and their products interact can illuminate new paths for treating complex diseases, paving the way for targeted therapies.
- Impact of Evolution on RNA: Investigating how evolutionary pressures shape RNA and its functionalities can yield insights into the adaptability and resilience of various organisms.
Through these avenues, we can enhance our understanding of fundamental processes while potentially crafting novel interventions that could transform the landscape of medicine and biotechnology as we know it.