The Role of Particle Detectors in Modern Science
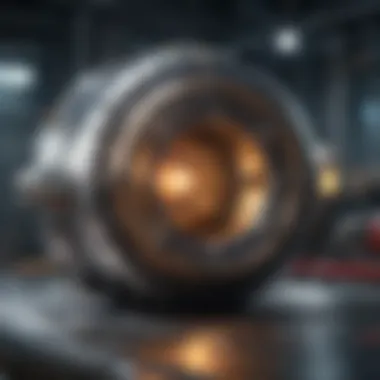
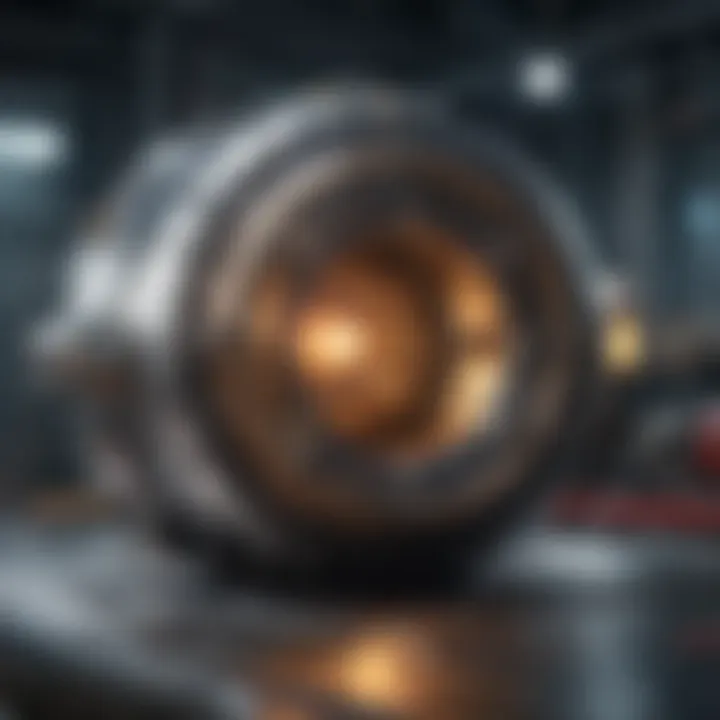
Intro
The world of particle detection is a cornerstone of modern science. Particle detectors are sophisticated devices that track and measure the properties of subatomic particles. Their development is essential in fields such as physics, astrophysics, and materials science. Understanding how these detectors function and their implications on scientific discovery is vital for researchers and students alike.
This article presents an in-depth analysis of particle detectors. It examines their design, methodologies, and the practical applications that stem from their usage. Furthermore, it delves into their critical role in identifying fundamental forces and particles of our universe.
Throughout this exploration, readers will gain insights into the collaboration between theoretical frameworks and technological advancements in particle detection. It is geared towards those looking to grasp both the nuances and the broader impact of these devices in contemporary scientific inquiries.
Methodologies
Description of Research Techniques
Particle detectors encompass a range of research techniques. These devices operate by observing the interaction between particles and a material medium. Common techniques include:
- Ionization: Particles ionize atoms in a detector medium, producing charged particles that can be measured.
- Scintillation: When particles interact with certain materials, they emit light. This light is then detected and analyzed.
- Cherenkov Radiation: This occurs when a particle travels faster than the speed of light in a medium, producing a characteristic light cone.
Each technique has unique advantages. For example, ionization detectors are widely used due to their sensitivity and reliability, while scintillation detectors excel in detecting low-energy particles.
Tools and Technologies Used
Particle detectors rely on advanced tools and technologies to enhance their capabilities. Some notable tools include:
- Silicon detectors: These provide high-resolution tracking of particles in various experiments.
- Wire chambers: These are essential for tracking charged particles in high-energy collisions.
- Photomultiplier tubes: They amplify the signal generated from light emissions in scintillation detectors.
Recent advances also involve digital signal processing and machine learning for better data analysis. This synergy between technology and particle physics signifies a major leap in precision and functionality.
"The evolution of particle detectors mirrors the rapid advancement of technology, showcasing how innovation fuels scientific discovery."
Discussion
Comparison with Previous Research
The impact of particle detectors on experimental physics opens extensive dialogue with historical research methods. In the past, earlier models such as cloud chambers and bubble chambers laid groundwork for current technologies. Techniques used then primarily relied on visual observation and limited data collection, whereas modern tools provide detailed, quantitative data.
The shift from analog to digital formats has transformed the speed and accuracy of data collection. This progression allows researchers to explore phenomena that were once deemed immeasurable.
Theoretical Implications
The advancement in particle detectors has significantly influenced theoretical frameworks within physics. For instance, the discovery of the Higgs boson at CERN's Large Hadron Collider was made possible due to sophisticated detector technologies. Such findings offer profound insights into the Standard Model and may prompt revisions or extensions of existing theories.
Prelude to Particle Detectors
Particle detectors are essential tools in modern science. They are crucial in various fields such as high-energy physics, astrophysics, and medical physics. At their core, these devices serve to identify and measure particles that are fundamental to our understanding of the universe and its underlying forces. The significance of particle detectors lies not only in their ability to reveal the properties of different particles but also in their capability to advance technological innovations.
The operation of particle detectors is based on the precise interactions that particles have with matter. Understanding these mechanisms is vital for improving detection methods and enhancing research outcomes. Various types of particle detectors have been developed, each suited for specific applications. Key examples include gas-based detectors, solid-state detectors, and liquid detectors. Each of these variants holds unique advantages that address different experimental needs.
One of the most important aspects of this discussion is the impact that particle detectors have had on scientific discovery. For instance, the detection of the Higgs boson was facilitated by sophisticated particle detectors in the Large Hadron Collider. This discovery not only confirmed long-standing theories in physics but also opened new avenues for exploration.
There are several factors to consider when discussing particle detectors:
- Design Complexity: Particle detectors often involve sophisticated designs that integrate various materials and technologies.
- Sensitivity and Resolution: A detector's ability to accurately identify particles depends on its sensitivity and resolution capabilities.
- Environmental Factors: Detectors can be influenced by external conditions, necessitating calibration and maintenance.
"Particle detectors not only elucidate the fabric of the universe but also inform the future of scientific inquiry."
Historical Context of Particle Detection
Understanding the historical context of particle detection is crucial in appreciating the advancements in physics and technology. It provides foundations for modern studies. Through examining the evolution of detection methods, we can grasp how early visions shaped current methodologies. This section sheds light on the critical milestones in particle detection, articulating how scientific inquiry evolved over time.
Early Innovations
The journey of particle detection began with rudimentary devices. Early innovations such as the cathode ray tube, invented by Johann Hittorf in the mid-19th century, marked significant progress. This device allowed scientists to visualize the behavior of cathode rays. This laid groundwork for future technologies.
Another pivotal invention was the Geiger-Müller counter, introduced by Hans Geiger and Walther Müller in the 1920s. It enabled researchers to detect and measure ionizing radiation effectively. These early tools gave insights into atomic and subatomic particles, and they sparked interest in further exploration. The simplicity and effectiveness of these agencies inspired a wave of experiments driving scientific curiosity.
Evolution Through the Decades
As the 20th century progressed, particle detectors evolved rapidly. The 1950s and 1960s saw the introduction of bubble chambers and spark chambers. These advancements enhanced the ability to track particles visually. Scientists could now observe interactions on a more meaningful scale, which contributed immensely to high-energy physics.
The advent of solid-state detectors in the 1980s represented another important leap forward. Devices like silicon detectors offered improved efficiency and precision. They revolutionized research in fields like high-energy physics and medical imaging.
In recent decades, advancements in digital technologies further improved data collection and analysis, making detectors more sophisticated. Innovations in computer science and signal processing enabled real-time analysis of data, increasing the throughput of experiments. This ongoing evolution reflects the dynamic interplay between theoretical developments and practical needs in the field of particle detection.
"The history of particle detection is a testament to scientific creativity, dedication, and the unyielding quest for knowledge."
Both early innovations and the evolution through the decades showcase the necessity of adaptive thinking in scientific communities. Equipped with historical insights, modern researchers can appreciate the challenges and triumphs that have shaped the tools of particle detection today. Understanding this context informs better practices and inspires future innovations.

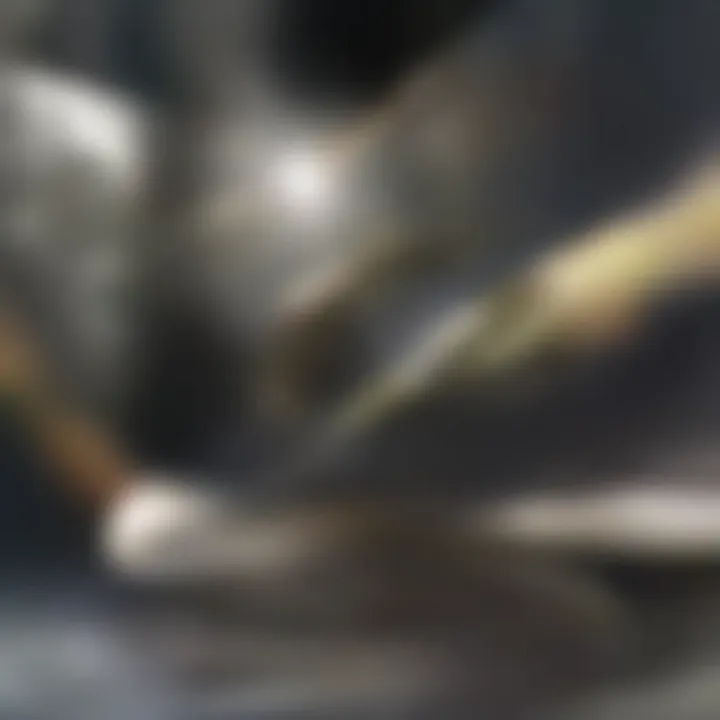
Engagement in interdisciplinary endeavors often benefits from this accumulated knowledge, illustrating the powerful connections across various fields. As we progress into future realms of detection, the history provides a compass guiding the next phases of discovery.
Principles of Particle Detection
The principles of particle detection form the foundational framework that underpins our understanding of how particle detectors operate. It is essential to grasp these principles to appreciate the broader impact that particle detectors have in scientific research. The effectiveness of a particle detector largely depends on its ability to identify and characterize particle interactions accurately. Understanding these principles also helps researchers design more sensitive and precise detectors.
Basics of Particle Interactions
Particles, whether they are electrons, protons, or more complex entities, constantly interact with one another and with various materials. These interactions can produce secondary particles or cause energy transfer, both of which are critical for detection. The basic principles behind these interactions include electromagnetic forces, weak nuclear forces, and strong nuclear forces.
When a particle passes through a detector medium, it can ionize the atoms, leading to the production of electron-hole pairs. This ionization is fundamental as it serves as the first step in the detection process. A thorough understanding of these basic interactions guides researchers in optimizing the design and operational method of different detectors, enhancing their sensitivity and accuracy.
Detection Mechanisms
Detection mechanisms are the critical components that allow particle detectors to interact with and measure particles. Three prominent mechanisms include scintillation, charge multiplication, and track reconstruction. Each mechanism has distinct characteristics, advantages, and limitations that make them suitable for specific applications.
Scintillation
Scintillation is the process where certain materials emit light in response to ionizing radiation. This light emission can be converted into an electrical signal, which is then processed to identify the properties of the incoming particle. One key characteristic of scintillation is its speed; the emitted light can be detected in real-time, making it a favored choice for many experimental setups.
The unique feature of scintillation detectors is their variety of materials that can be used to create scintillators, ranging from organic compounds to inorganic crystals such as sodium iodide. The versatility of these materials allows for tailored solutions based on required sensitivity and energy resolution. However, scintillation detectors may face challenges such as dependence on the specific material chosen and limitations related to photon absorption in thicker layers.
Charge Multiplication
Charge multiplication refers to the process that amplifies the detected signal by generating multiple charge carriers from a single interaction. This mechanism is particularly important in gas-based detectors, like Geiger-Müller tubes or proportional counters. A notable characteristic of charge multiplication is its ability to produce a significant output signal even from low-energy interactions, enhancing sensitivity.
The unique advantage of charge multiplication is its effectiveness in low-light conditions, which is crucial in experiments where high background noise might obscure signal detection. On the other hand, care must be taken in calibration, as the signal can saturate if too many interactions occur too quickly, leading to misreadings.
Track Reconstruction
Track reconstruction is essential for determining the trajectory of charged particles as they travel through a detector. This mechanism allows researchers to visualize and map out particle paths, which is vital for understanding complex interactions in collisions or decays. A key characteristic of track reconstruction is its reliance on precise spatial measurements to ensure accurate representations of particle paths.
Track reconstruction significantly contributes to high-energy physics experiments, such as those conducted at the Large Hadron Collider. The spatial resolution and the ability to correlate multiple readings allow scientists to infer particle masses and types. Challenges arise when dealing with overlapping tracks, which can complicate analysis. Despite this, advancements in detection technologies continue to improve reconstruction methods, offering greater accuracy and efficiency.
Understanding the principles of particle detection is crucial for advancing modern science and enhancing experimental capabilities across various fields.
In summary, the principles of particle detection, including the basics of particle interactions and detection mechanisms such as scintillation, charge multiplication, and track reconstruction, form the bedrock of particle detector functionality. Each mechanism offers unique insights and has distinct advantages that make it suitable for different experimental requirements.
Types of Particle Detectors
Particle detectors play a critical role in scientific discoveries. They help researchers observe particles that are too small for the naked eye. Understanding the types of detectors is essential to grasp how they contribute to modern science. Each type has its advantages and is suited for different applications. Identifying the right detector type is vital for the success of experiments.
Gas-based Detectors
Gas-based detectors operate by using gas as a medium to detect charged particles. The most common type is the Geiger-Müller counter, which detects ionizing radiation. When a particle passes through the gas, it ionizes atoms, creating electron-ion pairs. These ions then generate a measurable electrical signal.
This type of detector is often preferred for its simplicity and portability. They are used in various fields, including radiation monitoring and nuclear physics. Their capacity to measure low levels of radiation makes them valuable in safety applications. However, they have limitations, such as a lower energy resolution compared to solid-state detectors.
Solid-state Detectors
Solid-state detectors employ semiconductor materials to detect charged particles. Silicon detectors are a common example. When a charged particle interacts with the semiconductor, it creates electron-hole pairs. These pairs can then be collected to produce a signal.
The precision of solid-state detectors is high, making them essential in high-energy physics experiments. They offer better energy resolution, which allows researchers to distinguish between different types of particles. Advancements in solid-state technology have led to improved performance and reliability, crucial for experiments like those conducted at the Large Hadron Collider.
Liquid Detectors
Liquid detectors use liquids, often scintillating fluids, to detect particles. When a particle interacts with the liquid, it emits light, which is recorded by photodetectors. A significant advantage of liquid detectors is their capability to measure a wide range of particle energies.
These detectors are critical for neutrino detection and are employed in experiments focused on understanding fundamental particles. However, the need for special handling and their relatively complex setup can pose challenges.
Hybrid Detectors
Hybrid detectors combine features of different detector types. For instance, they may utilize solid-state and gas technologies. This approach allows for exploiting the strengths of each type while mitigating their weaknesses. Hybrid detectors are becoming increasingly popular in high-energy physics.
They can achieve better precision, and researchers can tailor them to specific experimental needs. Examples include advanced systems used at research facilities like Fermilab. Their flexibility makes them valuable in experiments that require high sensitivity and versatility, contributing significantly to ongoing research efforts.
In summary, understanding the types of particle detectors is essential for researchers. Each detector type serves unique purposes and fits distinct needs within the scientific community. This information is vital for making informed decisions in experimental design.
Research Applications of Particle Detectors
Particle detectors play a crucial role in various fields of scientific research. Their ability to observe and identify elementary particles leads to profound advancements in understanding the universe, forces at play, and their interactions. In this section, we will explore three primary areas where particle detectors have a significant impact: high-energy physics, astrophysics and cosmology, and medical physics.
High-energy Physics
High-energy physics relies on particle detectors to explore the fundamental components of matter. Facilities like CERN’s Large Hadron Collider utilize advanced detectors to observe particle collisions at unprecedented energy levels. These experiments test theoretical predictions and can lead to discoveries such as the Higgs boson, observed in 2012.
Particle detectors in this field provide crucial data regarding:
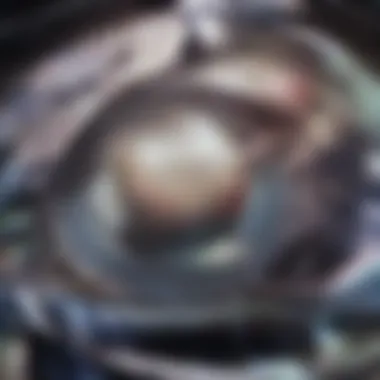
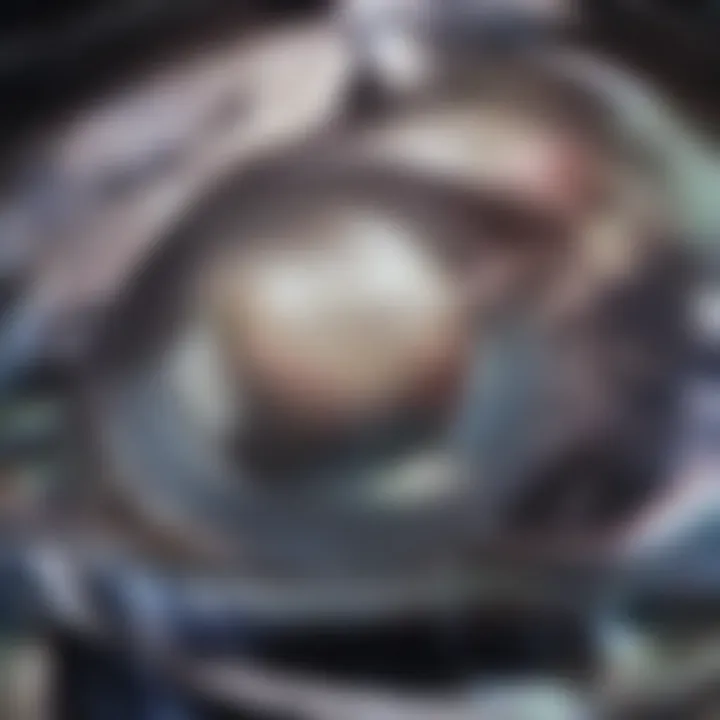
- The standard model of particle physics.
- The nature of dark matter and dark energy.
- Forces and interactions at the subatomic level.
Without sophisticated detectors, our understanding of particle interactions would remain limited. Their design and operational capabilities are tailored to reveal behaviors that occur at extremely short time scales and small distances. Thus, particle detectors equip researchers with the tools necessary to push the boundaries of known physics.
Astrophysics and Cosmology
In astrophysics, particle detectors enable the observation of cosmic phenomena. Neutrinos, for instance, are elusive particles that carry information about stellar processes and atmospheres far beyond our planet. Detectors such as Super-Kamiokande in Japan contribute to studies of solar neutrinos and supernovae events.
Researchers in this area benefit from:
- Enhanced understanding of the early universe.
- Insights into the life cycle of stars and black holes.
- Contributions to the field of cosmology, particularly in studying the cosmic microwave background radiation.
The integration of particle detectors within astrophysical research promotes interdisciplinary collaboration, merging physics, astronomy, and advanced engineering disciplines. Through these investigations, scientists can unravel mysteries of cosmic structure and evolution.
Medical Physics
In medical physics, particle detectors are utilized to enhance imaging technologies and treatment methods. For instance, positron emission tomography (PET) scans rely on the detection of gamma rays emitted from positron-electron annihilation events. This allows for detailed visualization of metabolic processes in the body.
Applications of particle detectors in medicine include:
- Cancer Diagnosis: Precise imaging can lead to earlier detection of tumors, improving treatment outcomes.
- Radiation Therapy: Particle therapy, including proton beams, delivers targeted treatment to tumors while minimizing damage to surrounding tissues.
- Safety Monitoring: Detectors ensure safe levels of exposure in medical imaging, protecting both patients and healthcare staff.
These advancements illustrate how particle detectors transcend traditional physics applications. Their integration into the health sector represents a vital exploration of fundamental principles applied for societal benefit.
As we move forward, it is clear that particle detectors will continue to reshape and redefine our research capabilities across multiple domains. Their functionality and impact mark a significant chapter in the evolution of scientific inquiry.
Technological Advancements in Particle Detection
The field of particle detection has seen significant innovations in recent years. These advancements are crucial as they allow scientists to explore the subatomic realm with greater precision and efficiency. The integration of modern technologies enhances the capability of detectors, ensuring they meet the demands of contemporary research. In this section, the focus will be on two main aspects: digital signal processing and integration with computing technologies.
Digital Signal Processing
Digital signal processing (DSP) has become a fundamental component in modern particle detectors. This technology is essential for converting analog signals into digital data that can be easily analyzed. The transition from analog to digital simplifies the interpretation of the data generated by the detectors.
Benefits of Digital Signal Processing include:
- Increased Sensitivity: DSP improves the ability to detect faint signals.
- Noise Reduction: Advanced algorithms can filter out background noise, ensuring clearer signal acquisition.
- Real-Time Processing: DSP allows for immediate analysis of signals, which is vital during experiments where timing is critical.
Research facilities, such as CERN, rely on DSP techniques to gather substantial data from collision events. These techniques also facilitate sophisticated data reconstruction processes, which are necessary for understanding the interactions of fundamental particles. With higher accuracy and speed, scientists can uncover insights that were previously unreachable.
"Digital signal processing acts as a bridge between raw data and meaningful scientific interpretation."
Integration with Computing Technologies
As particle detectors evolve, their integration with advanced computing technologies grows. This integration not only streamlines data collection but also enhances the analysis process. High-performance computing systems handle large volumes of data generated by the detectors effectively
Key aspects of this integration include:
- Big Data Analytics: New tools for data analysis allow researchers to process massive datasets. Techniques like machine learning are increasingly used to detect patterns in the data.
- Cloud Computing: This allows storage and processing power to be accessed remotely, offering flexibility and scalability.
- User-friendly Interfaces: Modern software platforms offer intuitive interfaces, making it easier for scientists to interact with complex datasets.
The synergistic effect of combining particle detectors with robust computing technologies fosters innovative discoveries. As these technologies continue to advance, one can expect new capabilities that further enhance the precision and capabilities of particle detection.
In summary, technological enhancements in digital signal processing and computing integration are reshaping particle detection. They enable scientists to achieve unprecedented accuracy and efficiency, ensuring the ongoing evolution of research in subatomic physics.
Challenges and Limitations
The exploration of particle detectors is a complex and multi-faceted subject. While these tools are revolutionary in transforming our understanding of the universe, several challenges and limitations must be acknowledged. These factors influence not only the effectiveness of detectors but also the outcomes of experiments that depend on their precision and reliability. Understanding these elements is crucial for researchers, engineers, and scientists working in particle physics.
Environmental Factors
Environmental conditions can significantly affect the performance of particle detectors. Variability such as temperature fluctuations, humidity, and electromagnetic interference can lead to inaccuracies in readings. For example, high temperatures may alter the material properties of detectors, affecting their sensitivity and response time. In a laboratory setting, shielding from external radiation and fluctuations in electromagnetic fields becomes essential to maintain accuracy in results.
Factors like cosmic rays also play a part in environments where high-energy physics experiments are conducted. Interactions with environmental radiation can cause background noise, leading to complications in distinguishing between genuine signals and noise. Thus, the designs of detectors often incorporate advanced environmental controls, but these add complexity and may increase costs.
Calibration and Maintenance
Regular calibration and maintenance are vital for ensuring the functionality of particle detectors. Without proper calibration, detectors can yield misleading results that might compromise entire experiments. The process involves checking and adjusting the sensitivity of the detectors to ensure they are responding accurately to particles. This process must be meticulous, as even slight deviations can lead to significant discrepancies in data.
Furthermore, ongoing maintenance is required to address wear and tear. Particle detectors often operate within extreme conditions, leading to potential failures over time. Regular inspections and adjustments help to prevent costly breakdowns and ensure that the detectors are in top working condition. Laboratories working on cutting-edge physics experiments often face the challenge of balancing rigorous maintenance with the desire for innovative experiments, making it essential to invest both time and resources into this area.
"In particle detection, the precision of measurements influences the integrity of research conclusions. Thus, overcoming challenges related to environmental factors and calibration becomes paramount for scientists."
Additionally, the integration of new technologies and software into detector systems can introduce new variables. Adapting these high-tech solutions necessitates feedback loops where researchers continuously refine their calibration processes to align with evolving standards and technologies.
Overall, while the challenges associated with particle detectors may seem daunting, addressing them head-on fosters advancements in the field, ensuring that these scientific instruments continue to contribute meaningfully to our understanding of the fundamental aspects of matter and energy.
Future Directions in Particle Detection
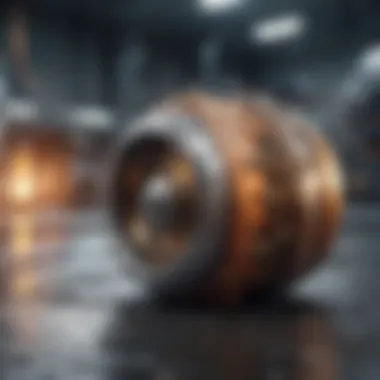
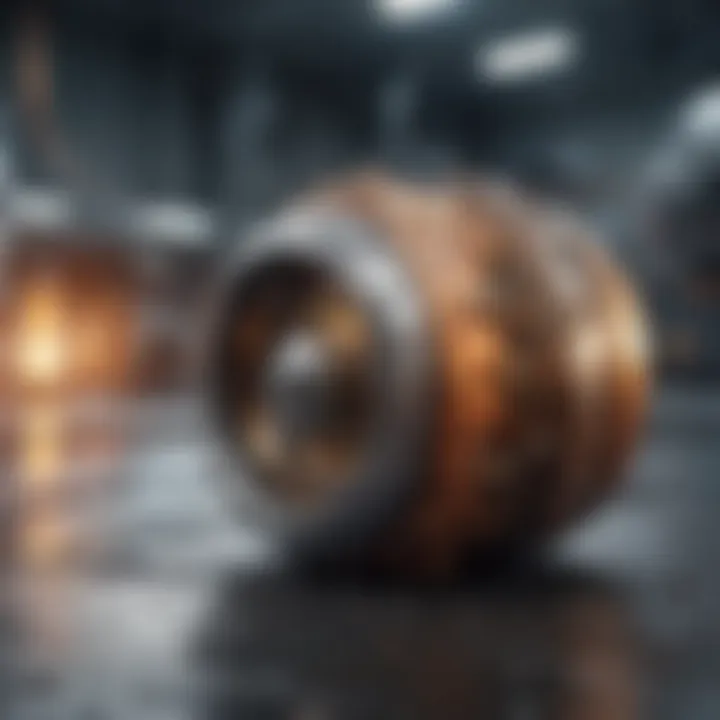
Particle detection is at a pivotal point in science. As technology evolves, so too does the capability of particle detectors. Future directions in this field hold great promise not just for physicists, but for a multitude of disciplines that rely on precise measurements and understanding of fundamental particles. This section will explore emerging technologies and potential scientific breakthroughs that are set to reshape our approach toward particle detection.
Emerging Technologies
New technologies are continuously emerging in particle detection. These innovations can lead to enhanced sensitivity and accuracy. For example, advancements in superconducting materials are playing a critical role in the development of devices like transition-edge sensors. These sensors operate at near absolute zero temperatures and can detect single photons, significantly increasing the sensitivity for low-energy particle interactions.
Nanotechnology is also making waves in this arena. By utilizing nanostructured materials, researchers are aiming to fabricate more efficient detectors. These detectors are lightweight and can potentially channel particles more effectively, improving both response time and resolution.
Additionally, machine learning is becoming an essential tool in processing data from detectors. Algorithms can analyze vast amounts of data in real time, identifying signals indicative of particle interactions much faster than traditional methods. This evolution not only expedites the research process but also helps in refining the detectors' functionality through feedback loops.
Potential Scientific Breakthroughs
As exciting as the technologies are, they herald possible breakthroughs in our understanding of the universe. One potential breakthrough lies in detecting neutrinos more effectively. Neutrinos are elusive particles. They interact only via the weak nuclear force, making them incredibly difficult to observe. Enhanced neutrino detectors might solve mysteries surrounding supernovae and the processes involved within the sun itself.
Moreover, advancements in particle detection can lead to better insights into dark matter. Dark matter remains one of the greatest enigmas of modern physics. Improved detectors could potentially capture dark matter interactions, providing hints about its properties and its role in the cosmos.
Beyond fundamental research, advancements in particle detection technologies can also spur innovations in medical imaging. For instance, PET (Positron Emission Tomography) scanners could become even more sensitive, aiding in the early detection of diseases such as cancer.
The future of particle detection promises to blend advanced technologies with groundbreaking scientific exploration, paving the way for discoveries that could change our understanding of both the micro and macro universes.
The intersection of these emerging technologies and potential breakthroughs illustrates the profound impact that particle detectors will have in shaping future scientific explorations. As collaboration across scientific disciplines grows, the possibilities become limitless.
Case Studies of Notable Experiments
The exploration of particle detectors finds substantial illustration through notable experiments in modern science. These case studies serve more than mere academic interest; they showcase the pivotal role these detectors play in advancing our understanding of fundamental physics. Through the lens of these experiments, we can appreciate the design, functionality, and ultimate impact of particle detectors, revealing how they have changed our conception of the universe.
The Large Hadron Collider
The Large Hadron Collider (LHC) stands as a monumental achievement in particle physics. Located at CERN, the LHC operates at unprecedented energy levels, facilitating collisions between protons at nearly the speed of light. Its motivation centers on exploring profound questions about the universe's origins and the nature of mass.
Importance of the LHC:
- Detection of the Higgs Boson:
The LHC was instrumental in confirming the existence of the Higgs boson in 2012, an essential particle responsible for giving mass to other particles. This discovery validated the Standard Model of particle physics, marking a significant advancement in scientific understanding. - Advanced Detector Technology:
The LHC employs several sophisticated detectors, including the ATLAS and CMS detectors. These complex systems utilize scintillation, charge multiplication, and advanced tracking systems to identify and measure particles produced during collisions. The integration of these detectors is critical for sifting through vast amounts of data, allowing scientists to isolate significant events. - Data Processing and Analysis:
The LHC generates a staggering amount of data daily, necessitating state-of-the-art digital signal processing and computing technologies. Researchers must apply intricate algorithms to filter, analyze, and interpret this data to identify potential new physics.
"The LHC is not merely a machine; it's a gateway to understanding the fundamental building blocks of the universe."
Neutrino Detectors
Neutrino detectors like IceCube and Super-Kamiokande highlight a different aspect of particle detection. Neutrinos are elusive particles, rarely interacting with matter. This property renders them challenging to study, yet they hold immense scientific potential for understanding cosmic events and fundamental forces.
Importance of Neutrino Detectors:
- Astrophysical Insights:
Neutrino detectors contribute significantly to astrophysics, providing insights into supernovae, black holes, and cosmic rays. For instance, IceCube has detected neutrinos from distant astrophysical sources, offering a new way to observe the universe. - Understanding Fundamental Physics:
These detectors enable the study of neutrino oscillation, a phenomenon where neutrinos switch between different types. This discovery has implications for the Standard Model and hints at the existence of new physics beyond current theories. - Interdisciplinary Approach:
The development of neutrino detectors emphasizes collaboration across various fields, uniting physicists, engineers, and data scientists. The multifaceted nature of these projects illustrates how particle detection stretches into diverse scientific domains.
In both case studies, the functionality and impact of particle detectors manifest in significant discoveries and breakthroughs within modern science. These experiments not only push the boundaries of our knowledge but also inspire new generations of researchers in their quest to unravel the mysteries of the universe.
Interdisciplinary Connections
The field of particle detection does not exist in isolation. Instead, it interweaves with various scientific domains, yielding significant benefits and enhancing the overall impact of research. Understanding the interdisciplinary connections is crucial for appreciating how particle detectors contribute to a wider array of scientific inquiries.
Collaboration with Other Scientific Fields
Particle detectors intersect with numerous branches of science. Physicists use detectors to study high-energy events, while biologists may utilize them for visualizing cellular processes. This cross-pollination of ideas facilitates groundbreaking discoveries.
Collaboration often leads to innovative techniques and methodologies. For instance, advancements in particle detection have influenced fields like environmental science, where detection methods are applied to monitor pollutants. Another example includes the application of scintillation detection in medical imaging. This not only improves our understanding of human physiology but also enhances diagnostic processes.
Effective collaboration also fosters the sharing of resources and expertise. Often, institutions will combine their knowledge to design cutting-edge detectors that serve diverse goals. Consequently, this results in a richer data set for analysis, enriching research outcomes considerably.
Impact on Technology Development
The advancements in particle detector technology ripple across various sectors. These developments often lead to improvements in unrelated technologies, thus having an extensive societal impact. As researchers refine detector design, they also contribute to advancements in data analysis techniques and miniaturization technologies.
Consider how semiconductor technology has evolved; innovations here have broader implications not just for particle physics but for electronics and computing. Similarly, the methods developed for signal processing in particle detectors can benefit telecommunications and information technology.
Moreover, the refinement of detectors has implications for safety and efficiency in industries like nuclear energy and medical radiation treatments. The evolution of these technologies showcases not just the symbiotic relationship between scientific inquiry and technological innovation, but also underscores the necessity for an integrated approach. As detectors improve, they generate a wealth of data that can lead to further research and innovation across various fields.
"The integration of different scientific disciplines can open up new horizons in research, allowing us to address complex challenges effectively."
In summary, the interdisciplinary connections illuminate the substantial role that particle detectors play beyond their immediate applications. Through collaboration with various fields and their influence on technology development, these detectors are instrumental in driving scientific progress.
Epilogue
Particle detectors have become a cornerstone of modern scientific inquiry. Their importance cannot be overstated, as they facilitate a deeper understanding of matter and energy at the most fundamental levels. Through this article, we examined various aspects of particle detectors, from their design to utilization in research, highlighting their intricate functionality.
One crucial element discussed is the versatility of different types of particle detectors. Each type, whether gas-based, solid-state, or hybrid, serves specific needs within various research fields. For example, the Large Hadron Collider employs advanced solid-state detectors to analyze high-energy particle collisions, providing insights into the fundamental structure of the universe.
Additionally, the article reviews the technological advancements that enhance the performance and precision of these detectors. Innovations such as digital signal processing and improvements in calibration techniques have significantly impacted the efficiency and accuracy of particle detection. These enhancements allow researchers to explore realms previously thought unattainable.
Moreover, the interdisciplinary connections made through particle detection research illustrate its broader impact. Collaborations with fields such as astrophysics and medical physics enable a transference of knowledge and technologies that bolster progress in both technology and fundamental science.
In terms of implications, the future of particle detection looks promising. Emerging technologies and methodologies will likely lead to revolutionary breakthroughs that could redefine our understanding of physical laws. This ongoing evolution underscores why particle detectors are not just instruments of measurement but essential components in the quest for knowledge.
In essence, as we continue to push the boundaries of scientific research, particle detectors will remain a vital tool in uncovering the mysteries of the universe, further solidifying their role in the advancement of modern science. Their contribution to elucidating the properties of fundamental particles and forces is invaluable, making them crucial for researchers, educators, and students alike.