Exploring the Process of Protein Synthesis in Cells
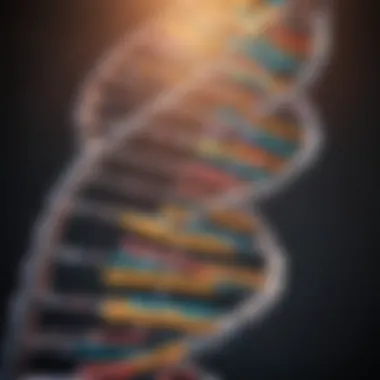
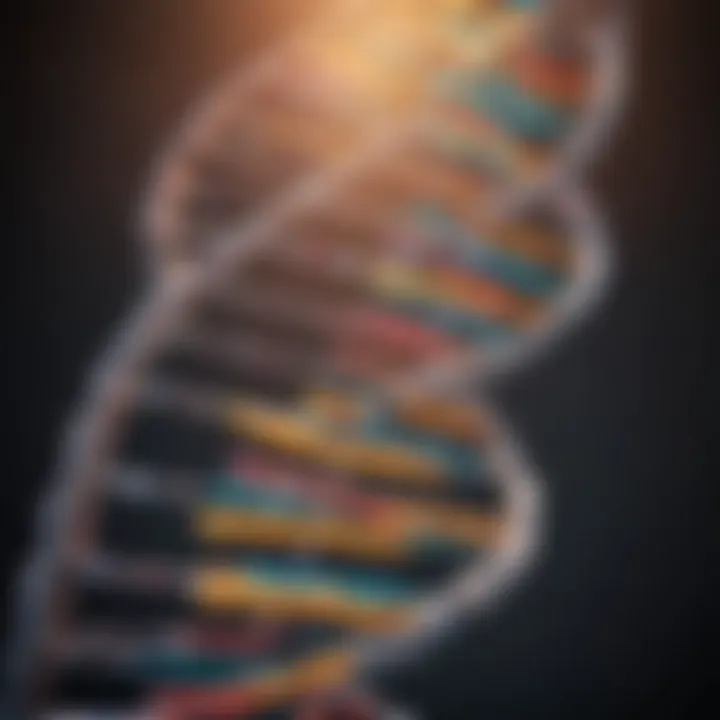
Intro
Protein synthesis is one of the fundamental processes that sustain life at the cellular level. Without it, cells would be unable to produce the proteins necessary for a variety of cellular functions, from structural support to enzymatic activity.
In the scope of this article, we will dive deep into the sequential steps of protein synthesis, which mainly include transcription and translation. Not only will we explore the molecular machinery involved, but we will also discuss why understanding this intricate process is essential, especially for those in the fields of biology and medicine.
The aim here is to break down the complicated world of proteins, showcasing how they are made from the genetic instructions coded in DNA. As we dissect each stage of the synthesis process, the significance of every component—be it RNA polymerase or ribosomes—will become clear.
Overview of the Protein Synthesis Process
The process of protein synthesis can be divided into two main stages:
- Transcription: The first step where DNA is converted into messenger RNA (mRNA).
- Translation: The subsequent step where the mRNA is read to build a protein.
Both stages involve various cellular components that work in unison, ensuring that the final product is not only vital but also correctly folded and functional.
In the following sections, we will explore methodologies, tools, and the theoretical implications behind these processes. This comprehensive exploration aims to shed light on the nuances of protein synthesis, making it easier for students, researchers, educators, and professionals to grasp its complexities.
Prelims to Protein Synthesis
Protein synthesis plays a pivotal role in the living world, influencing everything from the structure of cells to the function of enzymes. Just as a master chef follows a recipe to create a culinary masterpiece, cells utilize a detailed set of instructions encoded in DNA to synthesize proteins. This elaborate process is not just a biological phenomenon but a foundation of life itself.
Proteins are the workhorses of the cell, undertaking a variety of roles that are essential for survival. From catalyzing biochemical reactions to providing structural support, proteins enable cells to perform their functions effectively. Understanding the process of protein synthesis illuminates the intricate workings of cellular mechanisms and their implications in health and disease.
This article seeks to demystify the steps involved in protein synthesis, exploring the key components, mechanisms, and phases from the initial transcription of DNA to the final translation of mRNA into polypeptides. By delving into each aspect of this process, we can grasp the complexities and the sheer elegance of molecular biology—offering insights that are invaluable for students, researchers, educators, and professionals alike.
Definition and Importance
The term protein synthesis refers to the biological process through which cells construct proteins. It encompasses two main phases: transcription, where the information in DNA is converted into messenger RNA (mRNA), and translation, where this mRNA is read by ribosomes to assemble amino acids into a polypeptide chain. This mechanism is not merely a cellular curiosity; it underpins virtually every aspect of cellular function and organization.
Why is protein synthesis critical? Proteins govern countless cellular activities, including:
- Enzyme catalysis: Facilitating biological reactions.
- Structural support: Forming the framework of cells and tissues.
- Transport: Carrying molecules across cell membranes.
- Cell signaling: Participating in communication processes within and between cells.
Disruptions in protein synthesis can lead to severe consequences, manifesting in diseases such as cancer, cystic fibrosis, and various genetic disorders. Thus, understanding this process is crucial not only for biological research but also for the development of therapeutic interventions.
Overview of Cellular Functions
The synthesis of proteins operates at the heart of cellular functions. Every cell in an organism relies on this process to fulfill its roles, contributing to the complex interplay of life.
- Cell Growth and Repair: Proteins are essential for cellular growth and the repair of tissue damage. Without efficient protein synthesis, organisms would struggle to maintain homeostasis and adapt to environmental changes.
- Regulation of Metabolism: Enzymes, which are a type of protein, regulate biochemical pathways. They speed up reactions essential for metabolism, thereby ensuring that cells produce energy and synthesize compounds necessary for life.
- Immune Response: Proteins such as antibodies play a crucial role in the immune system, identifying and neutralizing pathogens. A well-functioning protein synthesis mechanism is fundamental for mounting a robust immune response.
In summary, the process of protein synthesis is intertwined with the very essence of cellular activity, shaping not just individual cells but also entire organisms. Each detail, from the specific roles of mRNA to the functions of ribosomes, reveals the sophistication of biological systems, an awe-inspiring dance of molecular interactions.
Key Components in Protein Synthesis
Understanding the key components involved in protein synthesis is crucial for grasping how proteins are created within living organisms. Every aspect of this intricate process relies on several essential molecules and structures that work together seamlessly, similar to a finely orchestrated symphony. Without these components, the entire operation crumbles.
DNA and RNA Overview
Both DNA and RNA play pivotal roles in the journey of protein synthesis. At the heart of the process lies DNA, the repository of genetic information. Structured like a twisted ladder, or a double helix, it holds the instructions necessary for building proteins. Each rung of this ladder represents a nucleotide, which consists of a sugar, a phosphate group, and one of four nitrogenous bases: adenine, thymine, cytosine, or guanine.
In contrast, RNA serves more as a messenger or intermediary. RNA is usually single-stranded and utilizes uracil in place of thymine. One of the crucial forms of RNA is messenger RNA (mRNA), which transcribes the genetic code from DNA and carries it out of the nucleus to the ribosomes, where proteins are made.
"The relationship between DNA and RNA is often described as that of a blueprint and a contractor; the blueprint outlines the entire design while the contractor builds according to those plans."
Ribosomes: The Protein Factories
Ribosomes could be considered the true workhorses of protein synthesis. These cellular structures, made up of ribosomal RNA (rRNA) and proteins, act like factories that translate the nucleotide sequence of mRNA into a sequence of amino acids— the building blocks of proteins. Ribosomes can be found floating in the cytoplasm or attached to the endoplasmic reticulum, forming the rough ER, which adds an extra layer of functionality by processing proteins.
When ribosomes assemble to begin translation, they do so in a meticulous manner. They read the mRNA strand in sets of three nucleotides, known as codons. Each codon corresponds to a specific amino acid, which will subsequently be linked together to form a polypeptide chain, ultimately folding into a functional protein. It’s kind of like a construction team reading plans and working together to put up a building piece by piece.
Amino Acids: The Building Blocks
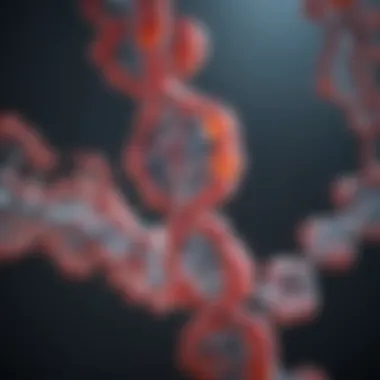
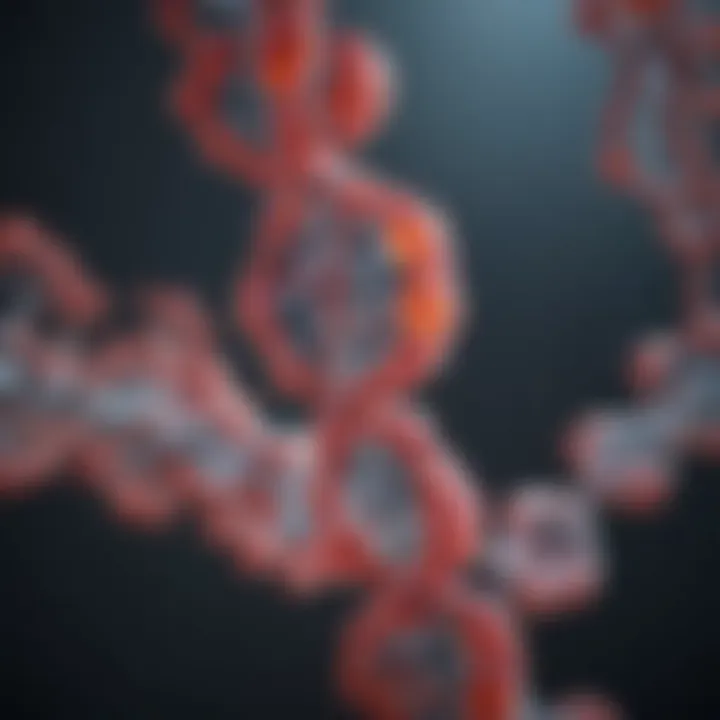
Amino acids deserve their moment in the spotlight, as they are the fundamental units that constitute proteins. There are 20 different types of amino acids, each possessing unique side chains that dictate their chemical properties. These amino acids connect through peptide bonds to form long chains that fold into intricate shapes, determining the protein’s function within the cell.
To make the right protein, the cell must balance the types and numbers of amino acids it uses. This is where the beauty of precision comes into play; a misstep in this elaborate dance can lead to a dysfunctional protein, which might disrupt normal cellular operations.
In summary, these key components—DNA, RNA, ribosomes, and amino acids—work in tandem to ensure that proteins are synthesized accurately and efficiently. Understanding their roles provides vital insights into not only biology but also medical research, biotechnology, and other scientific fields. The complex interplay among these elements exemplifies life at its most fundamental level.
The Transcription Process
The Transcription Process is a critical step in the broader narrative of protein synthesis. It acts as the bridge connecting the genetic code in DNA to the creation of proteins, serving as a blueprint necessary for subsequent stages like translation. Understanding this phase is fundamental for grasping how genes are expressed and how their codes are decoded into functional proteins within the cell. It offers us insights into various biological mechanisms, from cellular growth and replication to responses to environmental stimuli. As we delve deeper, we will explore how each step contributes to the overall efficiency and integrity of cellular functions.
Initiation of Transcription
Initiation is the first signal in the transcription dance. This process begins when RNA polymerase enzyme arrives at a specific sequence of DNA known as the promoter. Picture a conductor raising a baton; this moment marks the start of a complex symphony of molecular activities. Here, transcription factors play a crucial role, guiding the RNA polymerase to the right spot, much like navigators charting a course through unknown waters. Once the RNA polymerase is positioned accurately, it unwinds a small section of the DNA, making the corresponding DNA base pairs available for copying.
Elongation Phase
The elongation phase is where the real magic happens. After initiation, RNA polymerase starts to read the uncoiled DNA, synthesizing a complementary strand of RNA. The beauty of this step lies in the specific pairing of nucleotides: adenine pairs with uracil, and cytosine with guanine. The enzyme moves along the DNA, elongating the RNA strand by adding nucleotides one by one. This process is rapid, as many RNA molecules can be synthesized simultaneously from a single DNA template, maximizing efficiency.
Termination and mRNA Processing
Once the RNA polymerase has traversed the entire gene, it's time for termination. The process typically concludes when the polymerase encounters a terminator sequence. This is a well-orchestrated stop, signaling the end of transcription.
Following termination, mRNA undergoes several processing steps that are pivotal for its maturation and functionality in translation.
Splicing
Splicing is like editing a film; it removes unnecessary sequences known as introns from the pre-mRNA, while exons remain, forming the final editing cut that will be used in translation. This selective removal serves a dual purpose: it conserves cellular resources and ensures a streamlined message reaches the ribosomes. The key characteristic of splicing is its precision; an improperly spliced mRNA could lead to dysfunctional proteins, potentially causing significant cellular issues. Because of its adaptability, splicing is crucial, and it shows the intricate balance cells maintain between efficient gene expression and resource management.
Capping
Capping is another essential processing step that involves adding a modified guanine nucleotide to the 5' end of the mRNA strand. This cap acts similarly to an envelope that helps protect the mRNA from degradation by exonucleases, enzymes that would otherwise break it down. The unique feature of the cap is its role in ribosome recognition during the translation process, enhancing the efficiency of protein synthesis. Without this vital addition, the mRNA would be more likely to be targeted for destruction before it could fulfill its purpose.
Polyadenylation
Finally, polyadenylation adds a tail of adenine nucleotides at the 3' end of the mRNA transcript. This poly(A) tail increases the stability of mRNA and facilitates its export from the nucleus into the cytoplasm. Importantly, this tail also plays a role during translation; it interacts with proteins that help initiate protein synthesis. The key characteristic of polyadenylation is its ability to significantly enhance mRNA's lifespan and translational efficiency, making it a fundamental and beneficial feature in the entire transcription process.
"The transcription phase isn’t merely a preparatory step; it's a vital choreography that sets the stage for life's symphony of protein synthesis."
The Role of mRNA in Translation
Messenger RNA, commonly known as mRNA, serves a pivotal function in the overall framework of protein synthesis. Its primary purpose is to convey genetic instructions from DNA to the ribosome, an intricate structure where protein formation transpires. Without mRNA, the code inscribed within DNA might as well be a locked book, its secrets forever untold. Thus, the role of mRNA is not just instrumental; it’s the linchpin ensuring that proteins, which perform a vast array of essential functions in living organisms, are synthesized correctly.
The translation of mRNA into lifelong proteins marks an essential step in cellular machinery. mRNA works closely with ribosomes and transfer RNA (tRNA) to stipulate which amino acids will be strung together in a specific sequence to form a protein. This tightly-knit process unveils the complex choreography of molecular interactions that underpin life itself.
Structure of mRNA
The structure of mRNA is designed for efficiency and fidelity in protein synthesis. It’s a single-stranded molecule which often assumes a linear shape, allowing it to carry genetic information from the nucleus to the ribosomes in the cytoplasm. However, this linearity is not without its nuances; mRNA possesses distinct regions that contribute to its functionality. Essential components include:
- 5' Cap: This modified guanine nucleotide protects the mRNA from degradation and plays a role in ribosome binding.
- Coding Sequence: The codons that specify the amino acid sequence of the protein.
- 3' Poly-A Tail: A stretch of adenine nucleotides that enhances stability and assists in the export of mRNA from the nucleus.
This sophisticated structure enables mRNA to serve not only as a messenger but also as a regulatory element in various cellular processes. For instance, the length of the poly-A tail can impact the stability of mRNA, affecting how long it remains available for translation.
mRNA Codons and Amino Acids
Once mRNA is translated, the codons—the fundamental units of the genetic code—play a crucial role in defining the sequences of amino acids. Each codon consists of three nucleotides, which correspond to specific amino acids. Here's a glimpse into why these codons are so important:
- Each one acts like a VIP ticket, granting access to the assembly line of protein synthesis.
- Exceedingly precise, codons ensure that the correct amino acids are incorporated into the growing polypeptide chain.
- Importantly, if there's a mismatch, the end product might go haywire, which might lead to malfunctioning proteins.
The special relationship between codons and amino acids underpins the entire translation process. For example, the codon AUG not only signifies the start of protein synthesis but also codes for the amino acid Methionine, setting the groundwork for the remainder of the polypeptide chain.
In summary, mRNA is not just a simple messenger; it’s a complex structure loaded with vital information that, when translated, orchestrates the production of proteins crucial for life. An understanding of its role, structure, and the interplay between codons and amino acids brings us one step closer to appreciating the intricate phenomena of protein synthesis in living organisms.
The Translation Process
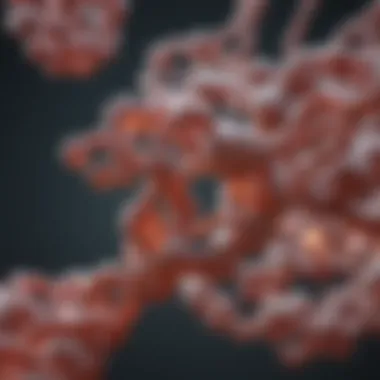
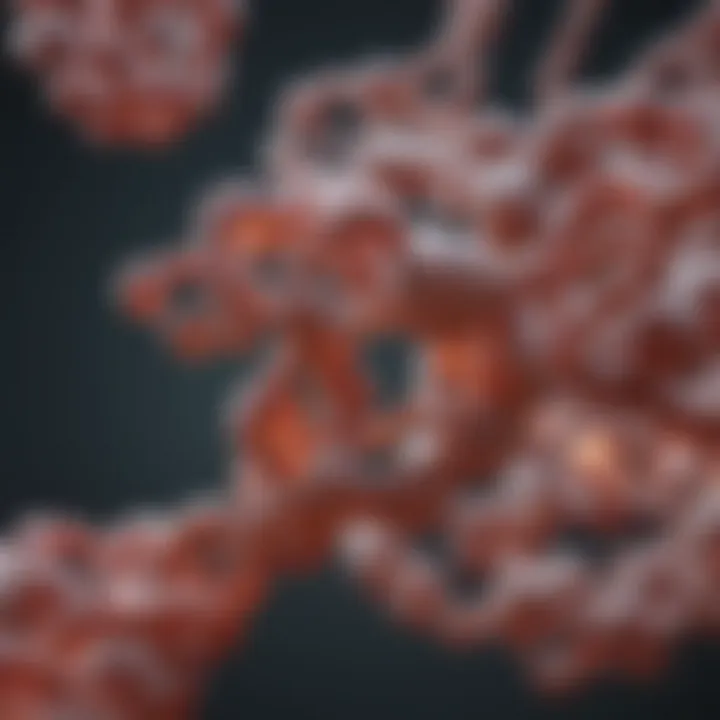
The translation process is a crucial step in protein synthesis, where the ribosomes assemble proteins according to the sequence of the messenger RNA (mRNA) transcript. It plays a significant role because it details how the genetic code in mRNA is transformed into the functional proteins that are vital for cellular function. This entire process is not just a mechanical conversion; it showcases the collaboration of various molecular components, each with its unique role.
In this part of the article, we will delve into the three main phases of translation: initiation, elongation, and termination. These phases highlight the dynamic interactions and processes that underscore the production of proteins. Understanding translation helps to appreciate how genetic information is utilized within cells, making it fundamental for both students and seasoned researchers.
Initiation of Translation
Ribosomal Assembly
Ribosomal assembly marks the starting point of translation, where the large and small ribosomal subunits come together on the mRNA strand. The ribosome itself is an intricate blend of ribosomal RNA (rRNA) and proteins, making it unique in its structure and functionality. One key characteristic of ribosomal assembly is its energy-dependent nature; it requires GTP hydrolysis to facilitate the joining of these subunits.
This assembly process is crucial because it sets the stage for accurate translation. Without proper ribosomal assembly, the whole mechanism can falter. Assembly ensures that the ribosome is appropriately positioned at the start codon of the mRNA, which is vital for the correct reading frame of the genetic code.
One of the unique features of ribosomal assembly is its ability to gradually adjust to various mRNAs. This flexibility allows for a broad range of proteins to be synthesized, contributing to cellular versatility. However, if the assembly goes awry, it can lead to dysfunctional proteins, which might result in various diseases.
Start Codon Recognition
Start codon recognition is the next important phase in the initiation of translation. This process specifically identifies the AUG codon in mRNA, which signifies the beginning of protein synthesis. The identification of the start codon is crucial because it determines where translation begins, thus influencing the entire downstream protein structure.
A key characteristic of this recognition is the involvement of initiator tRNA, which carries the first amino acid, methionine. The interaction between the tRNA and the AUG codon is highly specific, ensuring precision in the process. By recognizing the correct start codon, the translation machinery ensures that the resultant protein is synthesized correctly from the get-go.
In terms of unique features, start codon recognition is sensitive to the surrounding nucleotide context. This aspect adds a layer of complexity, as it requires the optimal sequence nearby the start codon to enhance recognition efficiency. This sensitivity may lead to advantages or disadvantages, contingent upon the presence of regulatory elements that might influence the translation efficiency.
Elongation of the Polypeptide Chain
tRNA Functionality
tRNA functionality is an essential element in the elongation phase, where transfer RNA molecules bring amino acids to the ribosome according to the codon sequence on the mRNA. Each tRNA is coupled with its respective amino acid at one end, while the anticodon at the other end base-pairs with the codon of the mRNA.
A key characteristic of tRNA functionality is its adaptability; these molecules can recognize multiple codons that correspond to the same amino acid due to the degeneracy of the genetic code. This adaptability ensures a smooth and efficient translation process, allowing cellular operations to run without a hitch.
One unique feature is the process of charging tRNA, which involves the enzyme aminoacyl-tRNA synthetase. This enzyme catalyzes the attachment of an amino acid to its corresponding tRNA, ensuring accuracy and efficiency in protein synthesis. Although charging is efficient, any mistakes in tRNA selection can lead to faulty proteins, which might impair cellular functions.
Peptide Bond Formation
Peptide bond formation is where the magic happens in elongation; this is the actual chemical process that links amino acids together to form a polypeptide chain. Once the tRNA occupies the ribosomal site, the ribosome facilitates the formation of a covalent bond between the amino acids attached to adjacent tRNAs.
The primary characteristic of peptide bond formation is its reliance on the ribosomal peptidyl transferase activity, a function intrinsic to the rRNA, highlighting the ribosome's role as a ribozyme. This enables it to catalyze the peptide bond without needing proteins.
A unique feature of peptide bond formation is that while it seems straightforward, it requires precise spatial and kinetic conditions. It’s a tightly regulated process, with numerous checkpoints to ensure that the right amino acids are linked in the correct order. If errors occur in this chain, it could lead to an entirely different protein, which can be problematic for the organism.
Termination of Translation
Stop Codons
Stop codons serve as the signal for the ribosome to terminate translation. These codons—UAA, UAG, and UGA—do not code for any amino acids, rather they indicate to the ribosomal machinery that the polypeptide chain is complete. The recognition of stop codons is critical, as it prevents unnecessary extension of the protein chain.
A major characteristic of stop codons is their specificity and universality across nearly all organisms; this highlights an evolutionarily conserved mechanism within the genetic code. Their presence guarantees an efficient end to the protein synthesis process.
In terms of unique features, the presence of release factors that recognize the stop codons greatly benefits the termination process. They bind to the ribosome, facilitating the release of the newly synthesized polypeptide chain. However, errors in stop codon recognition can result in the creation of longer proteins, potentially leading to malfunction.
Release Factors
Release factors are important players in the termination of translation. When the ribosome encounters a stop codon, these factors promote the release of the completed polypeptide chain from the tRNA. This action is vital as it clears the ribosome for future rounds of translation, ensuring that protein synthesis can continue unobstructed.
A key characteristic of release factors is their ability to mimic tRNA, allowing them to efficiently bind to the ribosome at stop codons. This mimicry is beneficial for the translation process as it fosters the precise termination of protein synthesis.
This characteristic brings unique advantages; for instance, it allows cells to effectively manage resources by clearing the ribosome after each translation cycle. However, an excessive or insufficient presence of release factors can either stall protein synthesis or induce premature termination, respectively.
Understanding the intricacies of the translation process enhances our grasp of molecular biology, sheds light on possible intervention points for therapeutic research, and underpins the very fabric of life's molecular events.
In closing, the translation process is rich with complexity and nuance. Each phase—the initiation, elongation, and termination—plays a vital role, contributing to the overall understanding of how proteins are synthesized. Recognizing these steps offers insights not only into basic biology but also into potential advancements in biotechnology and medicine.
Post-Translational Modifications
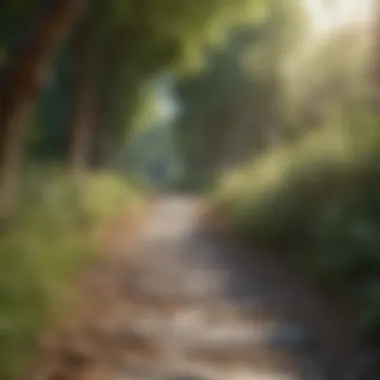

Post-translational modifications (PTMs) play a crucial role in shaping the functionality and lifetime of proteins. Once a protein has been synthesized, it rarely remains in its initial form. Various modifications occur that can significantly influence its activity, stability, and interaction with other molecules. PTMs are like added layers of complexity, allowing a single protein to participate in multiple pathways and fulfill various roles within the cell.
Why Modifications Matter
The significance of PTMs mustn’t be underestimated. They can determine a protein's fate in several ways, from altering its confirmation to specifying its location within a cell. For instance, modifications can signal whether a protein should be activated or deactivated. The implications are vast; in many diseases, the malfunctioning of PTMs results in impaired cellular signaling, leading to conditions like cancer or neurodegenerative diseases.
As we dive deeper, we can see that without these modifications, many proteins simply wouldn’t work as they should, almost like trying to drive a car without wheels.
Common Types of Modifications
- Phosphorylation
Phosphorylation is one of the most widely studied PTMs. It involves the addition of a phosphate group, typically to serine, threonine, or tyrosine residues on a protein. This modification is critical for numerous cellular processes, as it can either activate or inhibit enzyme activity. - Glycosylation
Glycosylation is another pivotal modification involving the addition of sugar moieties to proteins. This process can affect a protein's structure and stability, contributing to the biological activity of many glycoproteins. - Ubiquitination
Ubiquitination involves the attachment of ubiquitin, a small protein, to lysine residues on target proteins. This modification is crucial for marking proteins for degradation, especially those that are misfolded or no longer needed.
- Key Characteristic: Phosphorylation is highly reversible, which means that its effects can be quickly enacted and retracted, allowing for rapid responses to cellular signals.
- Why This Choice Matters: Its ability to rapidly change protein function makes it a popular choice in signaling pathways.
- Unique Features: The dynamics of phosphorylation involve complex networks of kinases and phosphatases that add or remove phosphate groups, respectively.
- Advantages/Disadvantages: While it allows for precision in regulating protein activity, excessive or insufficient phosphorylation can lead to serious diseases, including cancers.
- Key Characteristic: It’s almost an indicator of protein folding quality; properly glycosylated proteins tend to function more effectively.
- Why This Choice Matters: Glycosylation plays a critical role in cell-cell recognition, immunity, and protein solubility.
- Unique Features: Different types of sugars can be added, with branched versus linear structures impacting how a protein interacts in biological environments.
- Advantages/Disadvantages: While it can promote proper protein function, aberrant glycosylation is linked with various pathologies, including genetic disorders.
- Key Characteristic: It is often a signal for the proteasome to recognize the target protein and degrade it.
- Why This Choice Matters: Without ubiquitination, damaged or unnecessary proteins would accumulate and disrupt cellular processes.
- Unique Features: Ubiquitination can be a multi-step process resulting in polyubiquitination—a chain of ubiquitin molecules that can target proteins for destruction.
- Advantages/Disadvantages: Ubiquitination is vital for maintaining protein homeostasis; however, if deregulated, it can contribute to various diseases such as neurodegenerative disorders.
Post-translational modifications are not merely additional steps; they are fundamental alterations that dictate how proteins behave in a cellular environment.
In summary, PTMs are essential for the proper functioning of proteins. By modifying proteins after their initial synthesis, cells can adapt and respond to their constantly changing environments. Understanding these modifications reveals the intricate orchestration involved in life at the molecular level.
The Role of Chaperones in Protein Folding
Protein folding is a crucial step in the path from gene to function, and it’s where chaperones come into play as unsung heroes. These molecular helpers guide proteins through the winding roads of folding, ensuring they reach their final three-dimensional structure without getting tangled or misfolded. Understanding the role of chaperones is essential to appreciating the complexity of protein synthesis and the processes that maintain cellular health.
Importance of Proper Folding
The proper folding of proteins cannot be overstated in biological context. A protein’s function is intimately tied to its shape; just like a key fits into a specific lock, proteins interact only with their right partners when they are correctly folded. When proteins misfold, it can lead to a cascade of cellular failures that might drive diseases like Alzheimer’s or cystic fibrosis.
Chaperones recognize nascent or unfolding proteins and assist them in reaching their functional conformation. They provide a protective environment where these proteins can fold correctly. Notably, the efficiency of chaperones directly impacts cellular processes such as signaling, metabolism, and gene expression.
"Chaperones are the unsung heroes of the cellular world, tirelessly ensuring that proteins take the right shape to perform their essential functions."
To illustrate, without proper folding, proteins could form aggregates or clumps that aggregate and potentially become toxic to cells. Moreover, proteins that are functional can degrade more rapidly if misfolding occurs, leading to a loss of functionality. This makes the role of chaperones not just beneficial, but vital for the survival of the cell.
Heat Shock Proteins and Their Functions
Heat shock proteins (HSPs) form a specific class of chaperones that respond to stress conditions, such as heat, toxins, and oxidative stress. These proteins help in stabilizing other proteins under duress and prevent them from denaturing.
The functions of heat shock proteins include:
- Assistance in Folding: They assist newly synthesized proteins in folding correctly.
- Prevention of Aggregation: They prevent misfolded proteins from aggregating together.
- Refolding Damaged Proteins: In case a protein is already misfolded, HSPs can often refold these proteins back into their functional state.
- Targeting for Degradation: If a protein can’t be repaired, HSPs help mark it for degradation, thus preventing harmful accumulation in the cell.
HSPs are categorized into different families based on their molecular weight, including HSP70, HSP90, and small HSPs. Each family has distinct roles and operates under various stress conditions. Their importance has led researchers to keenly focus on these proteins both for understanding cellular responses to stress and for developing therapeutic interventions in various diseases.
By recognizing the significance of chaperones, we better appreciate the intricate dance of molecular biology that underpins life itself.
The End: The Complexity of Protein Synthesis
Protein synthesis is a sophisticated process that is not merely a series of steps, but a dynamic interplay of molecular players working in concert. Understanding this complexity is crucial, as it reveals not just how proteins are constructed but also their fundamental role in the biological landscape. From the moment a gene is transcribed into mRNA to the subsequent translation into a functional protein, each phase is riddled with opportunities for regulation, adaptation, and interaction.
Summary of Key Points
In summary, some key aspects we’ve explored include:
- Transcription and Translation: The diligent processing of genetic information is central. Transcription converts DNA into mRNA, while translation decodes this mRNA into proteins.
- Role of Ribosomes: These cellular machines are where translation happens, making them pivotal in the synthesis of proteins and their respective functions.
- Chaperones: These proteins assist in the proper folding of other proteins, emphasizing that synthesis isn’t the end; it’s just the beginning of a protein's lifecycle.
- Post-Translational Modifications: Just like a chef adds spices after cooking, proteins often require further modifications to become fully functional, highlighting the ongoing complexity beyond initial synthesis.
These elements are interdependent, further showcasing the nuances of protein synthesis.
Implications for Biological Research
Understanding the intricacies of protein synthesis has profound implications for biological research. Recognizing how errors in this process can lead to diseases underscores its importance in the medical and scientific communities. For instance, mutations that affect protein folding can result in conditions like cystic fibrosis or certain neurodegenerative diseases.
Additionally, advancements in biotechnology hinge on our grasp of protein dynamics. Techniques like CRISPR-Cas9 leverage our knowledge of proteins to edit genes, opening up vast possibilities in genetics research. The ability to manipulate and understand protein synthesis can lead to targeted therapies, improved drug design, and even enhance agricultural practices.
Moreover, as research continues to uncover the roles of chaperones and post-translational modifications, new avenues for therapeutic interventions will undoubtedly emerge. The landscape of proteomics is ever-evolving, demanding continuous inquiry into the steps we have mapped out in this article.
"Understanding proteins is to unravel the very enzymes of life itself."
The domain of protein synthesis represents a frontier in molecular biology, marrying structure with function, and precision with complexity. As we unpack these layers, we not only advance our scientific inquiry but also edge closer to unlocking the myriad mysteries of life itself.