Electrospinning Scaffolds: Principles and Applications
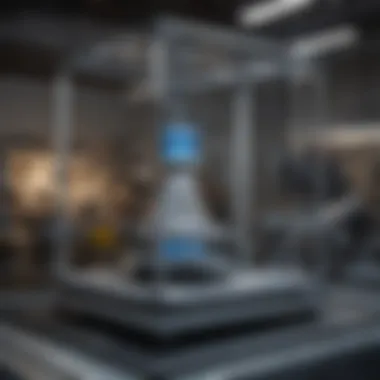
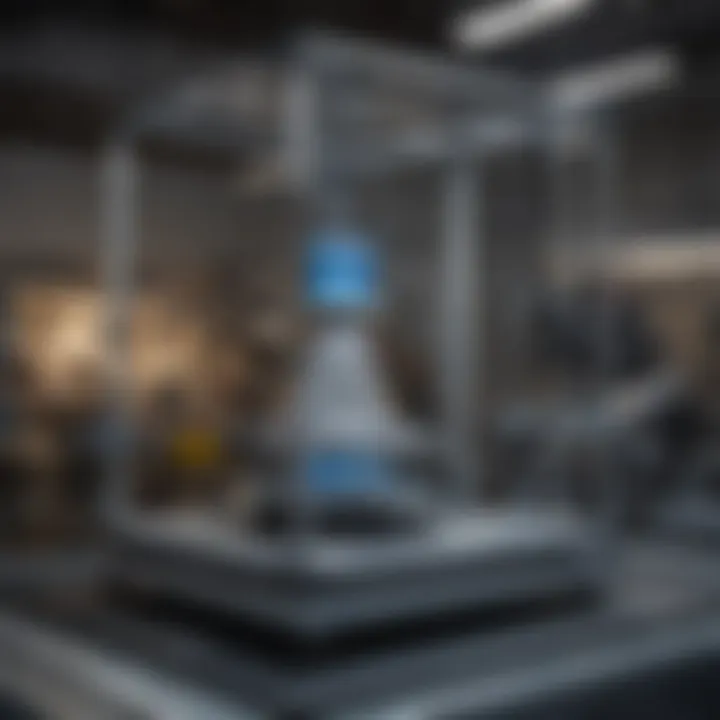
Intro
Electrospinning is an established method for producing nanofibers used primarily in scaffolds for tissue engineering. As health care continues to evolve, the demand for innovative techniques that assist in regenerative medicine increases. Electrospinning offers a versatile and efficient approach, enabling the creation of scaffolds with tailored properties. This introduction outlines the significance of electrospinning scaffolds in regenerative medicine, detailing their applications and innovations.
Overview of Electrospinning
Electrospinning is a process where a polymer solution is subjected to a high-voltage electric field. This causes the polymer to elongate and form fibers as it travels from a syringe to a collector. The fibers produced can mimic the structure of natural extracellular matrices, which is crucial for supporting cell adhesion and growth.
The exploration of electrospinning is essential for those involved in biomedical research. Understanding its principles aids researchers in creating more effective scaffolds that meet the diverse needs of tissue engineering. The efficiency of this method lies in its ability to manipulate the fiber diameter and porosity, critical factors that influence cellular processes.
Methodologies
Description of Research Techniques
Research into electrospinning involves a combination of experimental and theoretical methodologies. In vitro studies often assess the performance of these scaffolds in cellular environments. Researchers cultivate human cells on electrospun scaffolds to observe interactions. Key parameters include scaffold porosity, surface chemical composition, and fiber alignment.
These studies demonstrate how adjustments can positively or negatively impact cell behavior. For instance, increasing fiber density can improve cell attachment, whereas larger pore sizes might enhance nutrient transport, affecting cell proliferation.
Tools and Technologies Used
The production of electrospinning scaffolds employs various tools and technologies. Important instruments include:
- Electrospinning Setup: Comprising a syringe pump, high voltage power supply, and a collector.
- Characterization Equipment: Tools like scanning electron microscopy (SEM) are essential for analyzing fiber morphology, while tensile testing apparatus measure mechanical properties.
- Cell Culture Systems: These include incubators and bioreactors, crucial for conducting biological assays.
Such technologies ensure that the process of creating and analyzing electrospinning scaffolds is precise and replicable.
Discussion
Comparison with Previous Research
Previous studies in electrospinning have primarily focused on the materials used in scaffolds, such as polycaprolactone, polylactic acid, and collagen. These materials have varying properties, leading to different cellular responses. Recent research expands these materials to include bioactive components that promote healing and integration with surrounding tissues.
New studies increasingly employ novel techniques like multi-spinneret systems, enhancing the complexity and functionality of scaffolds. This advancement shows promise in improving scaffold performance significantly compared to traditional methods.
Theoretical Implications
The theories underpinning tissue engineering through electrospinning suggest an intersection of material science and biological interaction. Researchers propose that scaffolds not only serve a mechanical role but also play an active part in biochemical signaling. The future exploration of this interplay may uncover new, profound insights into the fundamental principles of tissue regeneration.
"Electrospinning scaffolds provide a platform for innovative approaches to tissue engineering, potentially revolutionizing regenerative medicine."
Prelude to Electrospinning
Electrospinning plays a pivotal role in the field of materials science, particularly in biomedical applications. It is a versatile and efficient method for producing nanofibers and scaffolds that mimic the extracellular matrix in tissues. Understanding electrospinning is crucial as it offers a unique solution to numerous challenges in tissue engineering, drug delivery systems, and wound healing applications. This section introduces the fundamental concepts of electrospinning, its significance, and how it serves as a vital technique in developing advanced materials.
Definition and Overview
Electrospinning is a process that uses an electric field to draw charged polymer solutions or melts into nanofibers. These fibers can be collected on a surface to form a non-woven mat. The process is notable for its simplicity and ability to produce high surface area-to-volume ratios. This characteristic is essential for applications in tissue engineering, where a large surface area can enhance cell adhesion and growth.
The basic setup of electrospinning includes a syringe with a polymer solution, a pump to control flow, a high-voltage supply, and a collector where fibers accumulate. The polymer solution is usually manipulated to reach a critical viscosity, allowing for effective electrospinning. Different parameters, such as voltage, distance, and solution properties, significantly influence the morphology of the fibers, which can be tailored for specific applications.
Historical Context
The electrospinning technique has its roots in the early 20th century. The first documented instance of electrospinning occurred in 1934 when F. P. Formhals described the process in a patent. However, it was not until the 1990s that the technique gained significant attention within the scientific community. The revival was largely due to advancements in polymer science and a growing interest in nanotechnology. Researchers began exploring electrospinning's potential in creating scaffolds for tissue engineering and drug delivery applications.
Notable developments in this field include the use of electrospinning for producing nanofibers from various materials, such as poly(lactic acid), polycaprolactone, and natural polymers like chitosan. Each of these materials offers unique properties that can be exploited in biomedicine. Today, electrospinning continues to evolve, with ongoing research aimed at improving techniques for better efficiency and scalability.
The Principles of Electrospinning
Understanding the principles of electrospinning is essential to appreciate its applications in scaffolding technologies. Electrospinning, as a manufacturing technique, relies on electrical forces to produce fibers from various polymer solutions. By controlling multiple variables, researchers can create scaffolds that mimic the architecture of natural tissues. This section serves to highlight the underlying mechanisms of electrospinning, which include the basic mechanisms involved, the role of electrical forces, and the mathematical models that help predict outcomes during the process.
Basic Mechanisms
At its core, electrospinning involves a simple yet fascinating setup that transforms a liquid polymer into a solid fiber. A polymer solution is typically contained in a syringe connected to a metallic needle. When a sufficiently high voltage is applied, various phenomena occur. The polymer solution is stretched into a fine jet, which then solidifies as it travels towards a grounded collector. The process primarily revolves around three critical phenomena: electrostatic repulsion, viscous drag, and surface tension.
Once the voltage is applied, electrostatic repulsion becomes dominant. The electrical field acts to overcome the surface tension of the solution, resulting in the formation of a Taylor cone at the needle tip. When the charge at the apex of the cone reaches a critical threshold, the jet is ejected towards the collector. This transformation leads to the elongation and thinning of the fiber, which is deposited as it dries in the air. The basic mechanisms of electrospinning thus lay the groundwork for efficient fiber creation in various applications.
Electrical Forces and Polymer Solutions
The interaction between electrical forces and polymer solutions is crucial for effective electrospinning. The viscosity of the polymer solution, its conductivity, and surface tension significantly influence the fiber formation process. High viscosity can hinder the formation of a stable jet, while low viscosity may lead to a coil-like structure rather than a linear fiber.
Moreover, the conductivity of the solution impacts the charge distribution within the polymer. Low conductivity can result in unstable jets, whereas high conductivity ensures a consistent and smooth jet ejection. In various studies, formulations of polymer solutions, such as Polycaprolactone and Polylactic Acid, have been optimized to achieve the desired mechanical properties while ensuring suitable electrospinning conditions. Careful selection of these parameters is essential for producing quality scaffolds, as they directly correlate to mechanical strength and porosity of the final product.
Mathematical Modelling
Mathematical modeling plays a significant role in understanding and predicting the outcomes of the electrospinning process. By employing models that incorporate physical parameters, researchers can simulate the behavior of electrospinning jets. Numerical methods based on fluid dynamics equations, such as the Navier-Stokes equations, are often used to explore the fluid flow and electrical field interactions in real time.
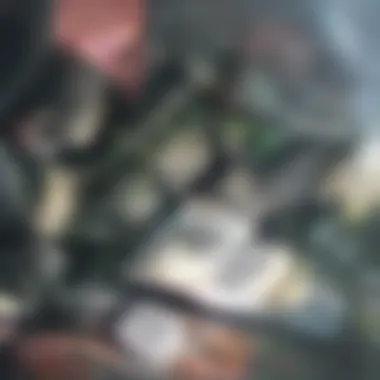
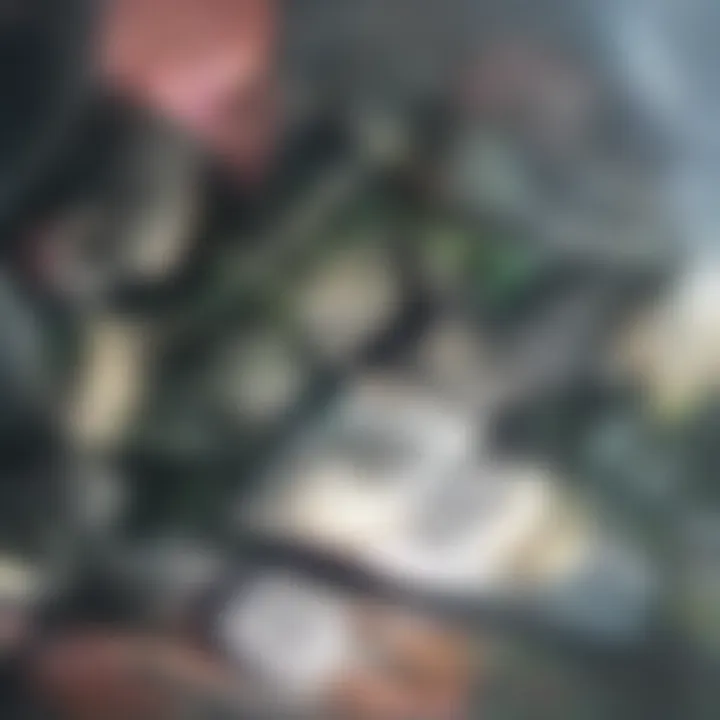
Several models have been developed to depict the viscosity, electric field strength, and surface tension effects during fiber formation. These models assist in refining the electrospinning process, allowing for better control over fiber morphology and alignment. Furthermore, advances in computational fluid dynamics enable researchers to iterate their designs efficiently, reducing both time and resource expenditure.
"The integration of mathematical modeling in electrospinning technology significantly enhances our understanding and control of the fiber fabrication process."
Materials for Electrospinning
Materials used in electrospinning play a crucial role in defining the physical and chemical properties of the resulting scaffolds. The choice of materials can significantly influence the mechanical strength, porosity, and biocompatibility of the fabricated nanofibers. This section discusses various types of materials employed in electrospinning, including natural polymers, synthetic polymers, and composite materials. Understanding these materials is essential for optimizing electrospinning techniques for specific applications in biomedical fields.
Natural Polymers
Natural polymers are derived from biological sources and are favored in many biomedical applications due to their innate biocompatibility and biodegradability. Common examples include collagen, chitosan, and gelatin. These materials not only facilitate cellular interactions but also mimic the extracellular matrix, promoting cell adhesion and growth.
Using natural polymers can yield scaffolds with properties that are similar to those found in native tissues. This similarity aids in cell proliferation and differentiation, making them ideal for tissue engineering. However, challenges like batch variability and limited mechanical strength need to be addressed.
Furthermore, the sourcing and processing techniques of natural polymers can affect the reproducibility of the scaffolds. Considering these variables is vital in their application, especially in clinical settings.
Synthetic Polymers
Synthetic polymers, such as polylactic acid (PLA), polycaprolactone (PCL), and polyethylene oxide (PEO), are extensively used in electrospinning due to their controllable properties. These polymers offer advantages like improved mechanical strength and tailored degradation rates, allowing for customization based on specific application requirements.
Unlike natural polymers, synthetic options provide consistency in composition, leading to reproducible results. This consistency is critical when designing scaffolds for drug delivery systems or load-bearing applications.
However, the biocompatibility of synthetic polymers can vary. To maximize their effectiveness, careful consideration of the polymer's surface properties is necessary. Modifications can be made through blending or copolymerization to enhance their properties and interactions with biological tissues.
Composite Materials
Composite materials combine two or more different components, harnessing the advantages of each to create superior scaffolds. By integrating natural and synthetic polymers, researchers can produce materials that possess enhanced mechanical and biological properties. For instance, combining chitosan with PCL yields a scaffold that is both strong and biocompatible.
These composites can also be functionalized with bioactive molecules to promote specific cellular responses. For example, incorporating growth factors into the scaffold design can significantly enhance cellular activities such as attachment and differentiation.
Despite these benefits, the complexity of fabricating composite materials can present challenges. Ensuring proper dispersion of components and achieving uniformity during electrospinning require careful optimization of processing conditions.
In summary, the choice of materials for electrospinning directly influences the functionality and applicability of the resulting scaffolds. Understanding the properties and behaviors of natural polymers, synthetic polymers, and composite materials allows for a more strategic approach in scaffold design.
By choosing the right material, researchers can address specific needs in tissue engineering, drug delivery, and other biomedical applications.
Electrospinning Techniques
The techniques used in electrospinning are crucial for producing scaffolds with specific properties and structures. Understanding these techniques can provide insight into how fiber characteristics can be modified to meet specific application needs. Each electrospinning technique has its advantages and limitations, making careful consideration essential for achieving desired results in terms of morphology and functionality.
Single Needle Electrospinning
Single needle electrospinning is the most basic and widely used method in the electrospinning process. This technique involves the use of a single syringe loaded with a polymer solution and a high-voltage power supply. The polymer is discharged from the needle tip as a jet, which elongates and thins due to the electrical forces applied.
One primary advantage of single needle electrospinning is its simplicity and ease of setup. It's particularly useful for small-scale experiments and fundamental research. However, the technique can have limitations in terms of production speed and scalability. Therefore, while suitable for laboratory settings, it may not be the best choice for industrial applications.
Coaxial Electrospinning
Coaxial electrospinning introduces a more complex structure by allowing two different polymer solutions to be electrospun simultaneously. Here, one solution is enclosed within another, providing unique benefits such as core-shell fiber configurations. This method is beneficial for encapsulating bioactive agents or drugs within a polymeric scaffold, which can enhance controlled release mechanisms in biomedical applications.
The coaxial technique also addresses issues related to mechanical properties and biocompatibility by allowing one polymer to provide strength while the other may serve as a biodegradable scaffold. Despite its advantages, coaxial electrospinning can be technically challenging and may require more precise control of the solutions' flow rates and the electric field.
Multijet Electrospinning
Multijet electrospinning represents an advanced alternative to the conventional single needle technique. In this method, multiple jets are generated from several needles simultaneously. This leads to a significant increase in fiber production rate, making multijet electrospinning a promising option for large-scale manufacture of nanofibers.
One key benefit of this technique is the ability to produce scaffolds with uniform fiber distribution over larger areas. This is particularly advantageous for applications where consistent properties are critical, such as in tissue engineering. However, like coaxial electrospinning, it may come with complexities such as higher initial costs and the need for more sophisticated equipment.
Multijet electrospinning’s ability to enhance fiber production efficiency makes it a strong contender for commercial applications in the future.
Overall, the approach selected for electrospinning can greatly influence the success of the scaffold's application. Choosing the right technique depends on specific requirements, including desired fiber morphology, mechanical strength, and functional properties.
Parameters Influencing Electrospinning
Understanding the parameters influencing electrospinning is crucial for optimizing the quality of scaffolds produced. These factors can directly affect the morphology and functionality of the nanofibers. Consistency in electrospinning outcomes results from careful consideration of these parameters, encompassing both the solution properties and processing conditions.
Solution Properties
The solution properties play a significant role in electrospinning. The viscosity of the polymer solution is perhaps the most critical factor. High viscosity solutions tend to produce thicker fibers, while low viscosity solutions may lead to bead formation instead of continuous fibers. Balancing viscosity is essential for achieving the desired fiber diameter and uniformity.
Other properties, such as conductivity, surface tension, and concentration, are also important. Conductivity affects the charge of the jet as it is ejected from the needle. Solutions with higher conductivity can produce more stable jets but may also risk unwanted instabilities. Surface tension can influence the formation of the Taylor cone, which is vital for initiating the electrospinning process. If the surface tension is too high, forming the cone may not occur, preventing successful electrospinning.
In addition, the concentration of the polymer affects the electrospinning process significantly. Optimum concentration allows for a smooth transition from a droplet to a jet. Too low concentration results in weak jets and bead formation, while too high can lead to difficulties in jet elongation and inadequate electric field interaction. Thus, tailoring these properties is crucial for successful fiber formation.
Process Parameters
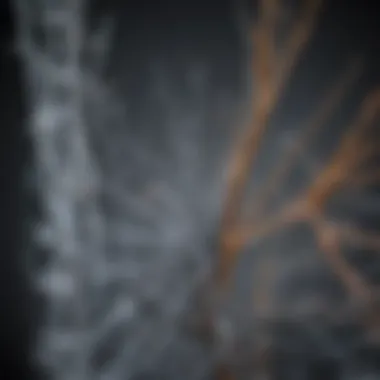
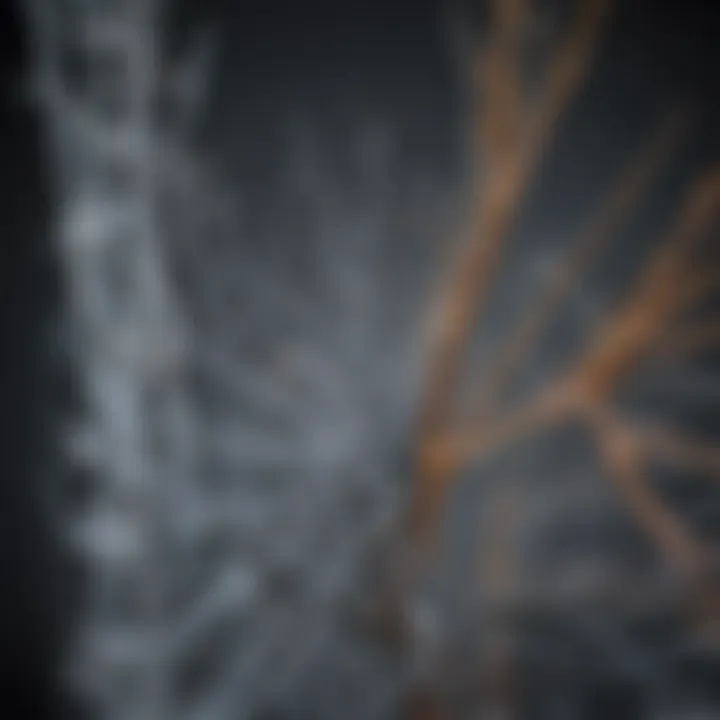
Process parameters encompass various settings applied during the electrospinning operation. These include voltage, distance between the needle and the collector, and the feeding rate. The voltage must be sufficient to overcome the surface tension of the polymer solution but not so high that it leads to unstable jet formation. A common range for electrospinning is between 10 to 30 kV, depending on the properties of the polymer solution.
The distance between the needle and collector is another influential factor. This distance can affect the drying process and the time the jet takes to travel before reaching the collector. Shorter distances may lead to thicker fibers due to less drying time, while longer distances may produce more uniform fibers with improved morphology.
The feeding rate affects the amount of solution ejected during electrospinning. A higher feeding rate can produce thicker and faster fiber deposits, but if too high, it can lead to droplet formation instead of a continuous fiber stream. Finding the right feeding rate is vital for creating uniform scaffolds.
Utilizing the right balance of solution properties and processing parameters is fundamental to achieving the desired outcomes in electrospinning technology.
In summary, the parameters influencing electrospinning, including solution properties and process parameters, are interdependent aspects that researchers must optimize. Understanding these elements allows for producing scaffolds with tailored properties necessary for diverse applications.
Characterization of Electrospun Scaffolds
Characterizing electrospun scaffolds is crucial for understanding their properties and functionality in various applications. This process involves evaluating the physical, mechanical, and biological characteristics of the scaffolds. A thorough characterization provides insights into the efficacy of the scaffolds in serving their intended purpose, particularly in the fields of tissue engineering and regenerative medicine. Key elements of this characterization include morphological analysis, mechanical properties, and biocompatibility testing. These factors directly influence cell behavior, scaffold integrity, and overall performance in biological environments.
Morphological Analysis
Morphological analysis plays a vital role in determining the structural features of electrospun scaffolds. This analysis not only examines the scaffold's fiber diameter, alignment, and porosity but also provides insights into how these features affect the scaffold's performance.
Scanning Electron Microscopy
Scanning Electron Microscopy (SEM) is a fundamental technique used for morphological analysis of electrospun scaffolds. SEM allows for high-resolution imaging of the surface of the scaffolds, revealing details that are essential for understanding their structure. One of the key characteristics of SEM is its ability to produce three-dimensional images by scanning the specimen with a focused beam of electrons. This technique is popular because it not only visualizes surface structures but can also provide spatial information about fiber orientation and interconnectivity.
A unique feature of SEM is its depth of field, which gives a greater range of focus compared to optical microscopy. This enables researchers to identify fine structural details that can influence properties like cell adhesion and fluid permeability. However, SEM requires sample preparation that can, at times, alter the original structure; this is a notable limitation when interpreting results.
Transmission Electron Microscopy
Transmission Electron Microscopy (TEM) is another essential method for morphological analysis of electrospun scaffolds. Unlike SEM, TEM involves transmitting electrons through ultra-thin specimens, leading to the creation of detailed images that show internal structures at the nanometer scale. This approach is particularly beneficial when examining the nanofiber's interactions and distribution in composite materials.
One key characteristic of TEM is its ability to provide insights into the internal morphology of fibers, which is often critical for understanding how structural properties affect biological responses. The main advantage of TEM lies in its exceptional resolution and the ability to visualize the internal architecture of scaffolds. However, the requirement for thin sections means that the preparation process is labor-intensive and might risk damaging the scaffold structure.
Mechanical Properties
Mechanical properties are essential for the functionality of electrospun scaffolds in biomedical applications. Understanding how scaffolds respond to stress and strain is critical in designing materials that can mimic the biological tissues they aim to replace or support. Key aspects include tensile strength, elasticity, and compressive strength. These properties determine how well the scaffold can support cellular activities while maintaining its structural integrity under physiological conditions.
Biocompatibility Testing
Biocompatibility testing assesses how well an electrospun scaffold integrates within biological systems. This evaluation often involves studying the interaction between the cells and the scaffold, focusing on parameters such as cell adhesion, proliferation, and differentiation. Biocompatibility not only ensures that the scaffold does not induce adverse immune reactions but also promotes the appropriate biological responses necessary for successful tissue regeneration. Proper testing regimens may include in vitro assays as well as in vivo studies to comprehensively assess the viability and acceptance of the scaffolds in biological environments.
Applications of Electrospinning Scaffolds
Electrospinning scaffolds play a critical role in a range of biomedical applications. Their unique structure, characterized by high surface area and porosity, makes them particularly suitable for various purposes in tissue engineering, drug delivery, and wound healing. Each application leverages the favorable properties of these fibrous scaffolds to address specific biological challenges.
Tissue Engineering
Tissue engineering is one of the most significant applications of electrospinning scaffolds. In this field, the primary goal is to create biological substitutes that can restore, maintain, or improve tissue function. Electrospun scaffolds provide a three-dimensional environment that mimics the natural extracellular matrix, which is essential for cell attachment and proliferation.
The fibrous architecture of these scaffolds promotes cellular penetration and nutrient diffusion. Furthermore, specific material choices, such as collagen, gelatin, or PLGA, can be tailored to enhance cell behavior, supporting differentiation into desired cell types. This characteristic is crucial for complex tissue constructs requiring multiple cell types.
In summary, using electrospinning for tissue engineering enables the design of tailored scaffolds that can improve cellular outcomes, making them invaluable in regenerative medicine.
Drug Delivery Systems
Electrospinning scaffolds are not only structural supports; they are also pivotal in drug delivery systems. The high surface area of nanofibers allows for loading a significant amount of therapeutic agents, such as proteins, peptides, or small molecules. Moreover, this method can control the release kinetics of the drug, providing sustained delivery over time.
Scaffolds can be designed with specific porosities and morphologies to manipulate drug release rates. This targeted approach ensures that medications are delivered where and when they are needed, enhancing therapeutic efficacy. The ability to incorporate multiple drugs into the electrospun fibers is an additional advantage, potentially addressing various conditions simultaneously.
In the context of disease treatment, electrospinning scaffolds emerge as a promising platform that combines localized treatment with sustained drug release, a critical factor for improving patient outcomes.
Wound Healing Applications
Wound healing remains a pressing concern in medical practice, and electrospinning scaffolds have demonstrated considerable potential in this area. The fibrous structure mimics the composition of natural skin and supports the healing process by providing a protective barrier against pathogens.
Electrospun scaffolds can also be functionalized to incorporate antimicrobial agents or growth factors that promote healing. This can minimize infection and enhance the regeneration of damaged tissue. Additionally, their flexibility and conformability allow these scaffolds to be applied to wounds of various shapes and sizes, ensuring a better fit and coverage.
In summary, the application of electrospinning scaffolds in wound healing not only enhances basic healing mechanics but also introduces therapeutic agents that can alleviate complications, showcasing their multifaceted role in modern medical solutions.
"Electrospun scaffolds bridge the gap between traditional methods and innovative biotechnologies, making them a cornerstone in the future of biomedical applications."
The various applications of electrospinning scaffolds underscore their importance in advancing medical science, particularly in areas where innovative solutions are critical for improving health outcomes.
Current Trends in Electrospinning Technology
The landscape of electrospinning technology is continually evolving. Recent trends reflect a growing emphasis on the enhancement of nanofiber production and the development of smart scaffolds. These advancements are critical for several reasons. They not only improve the functionality of electrospun scaffolds but also broaden the scope of their applications in biomedical fields. As researchers seek to create more sophisticated and responsive materials, understanding these current trends becomes essential.
Nanofiber Production Advances
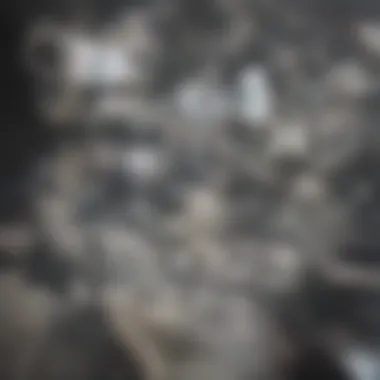
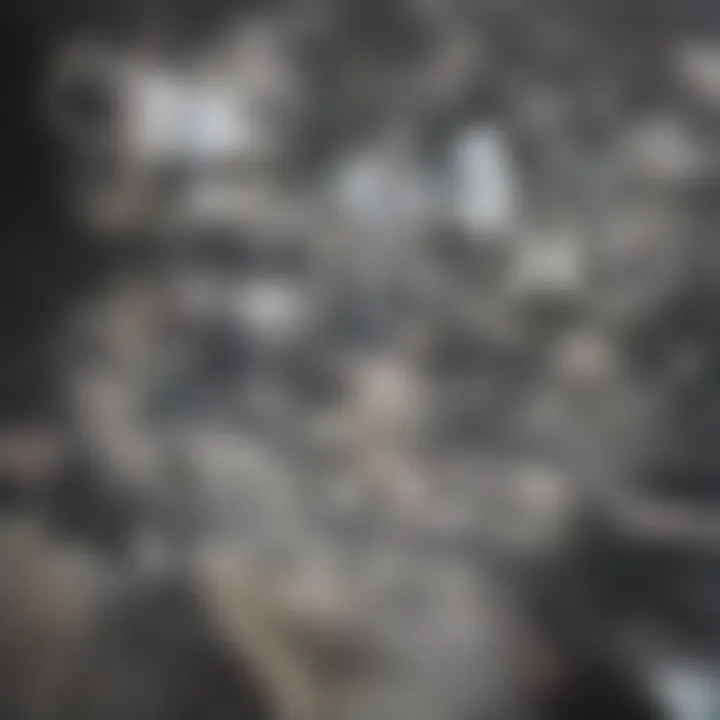
Nanofiber production technologies are witnessing significant advancements. These developments aim to increase production rates and improve the consistency of the fibers. A key trend involves the use of new electrospinning setups and strategies that enable continuous production. For example, multi-jet electrospinning is gaining attention because of its increased throughput. The technology allows multiple jets to operate concurrently, leading to a higher yield of nanofibers in a shorter time.
Furthermore, researchers are exploring the integration of novel materials that can improve the properties of the fibers. Enhanced polymers, such as polycaprolactone or biopolymers, offer better biocompatibility and mechanical strength. The refinement of these materials helps optimize the electrospinning process, creating fibers with precise characteristics suitable for varied applications in medicine and engineering.
"The adoption of advanced materials in electrospinning presents opportunities for novel applications in biomedicine."
Improving the efficiency and scalability of the electrospinning process presents challenges. One ongoing challenge lies in achieving uniformity in fiber diameter and morphology. Inconsistencies can lead to adverse effects on scaffold performance. To overcome this, researchers focus on controlling environmental factors, such as humidity and temperature during electrospinning.
Smart Scaffolds and Functionalization
Smart scaffolds represent a new frontier in electrospinning technology. These scaffolds can sense and respond to changes in their environment. Such responsive behavior enables them to perform specific functions, enhancing their applicability in biomedical contexts.
Functionalization is integral to the development of smart scaffolds. By modifying the surface of electrospun fibers, researchers can imbue them with desirable properties. For example, incorporating bioactive molecules can facilitate cell adhesion and promote tissue regeneration. Additionally, hydrophilic and hydrophobic properties can be precisely adjusted to control fluid interactions, further improving functionality.
Recent research highlights various functionalization techniques, including:
- Chemical modification: This approach alters the surface chemistry to enhance interactions with cells and growth factors.
- Physical modification: Techniques such as plasma treatment can create a rough or porous surface, which can improve cell attachment.
The integration of smart scaffolds into regenerative medicine holds potential for targeted drug delivery systems and advanced tissue engineering applications. As technology evolves, the interplay between electrospinning and smart materials will likely yield remarkable developments in scaffolds designed for personalized medicine and controlled therapeutic release.
Understanding these trends not only benefits academic research but also influences industrial applications, paving the way for innovations in healthcare solutions.
Challenges and Limitations
Understanding the challenges and limitations in electrospinning scaffolds is crucial for advancing this technology. These factors directly affect the efficacy and reliability of the scaffolds in practical applications. Identifying and addressing these challenges not only enhances scaffold quality but also broadens their applicability in fields like tissue engineering and drug delivery. An insightful exploration of these limitations offers critical perspectives on future research directions.
Scaffold Integrity and Stability
Scaffold integrity is of paramount importance in maintaining the structural and functional properties of electrospun scaffolds. The mechanical stability of these scaffolds is often compromised due to various factors. For instance, the porosity and fiber diameter play vital roles. A high porosity may facilitate cell infiltration, but it may also lead to decreased mechanical strength. Therefore, optimizing these parameters is essential for achieving a balance between functionality and stability.
Stability over time is another significant concern. Scaffolds may undergo degradation due to environmental conditions or biological interactions. For example, in a physiological environment, the presence of moisture or cells can result in the hydrolysis of certain polymers. Researchers must invest effort in selecting the right materials that can withstand specific physiological environments without losing integrity.
Maintaining scaffold integrity is critical in ensuring effective cell behaviors and overall function in tissue engineering applications.
Methods for testing stability, like mechanical testing or degradation studies, must be standardized. This ensures that data can be compared across studies, improving the reliability of findings in scaffold research.
Scalability Issues
Scalability remains a significant barrier in the widespread adoption of electrospinning technology. Many successful applications of electrospinning have been demonstrated at a small scale, but translating these successes to larger production levels involves several challenges. One significant factor is the consistency of fiber production when transitioning from a laboratory setting to an industrial environment.
For example, maintaining uniformity in fiber diameter and alignment is crucial for the scaffold's performance. Discrepancies in these parameters can lead to variations in mechanical and biological properties. Finding methods to control these variables without complicating the manufacturing process is essential for scalability.
Moreover, the availability and cost of raw materials can impact the feasibility of large-scale production. As demand for specific polymers increases, sourcing high-quality materials consistently becomes challenging. Thus, it is pivotal to explore alternative materials or methods that maintain performance while reducing costs.
The lack of robust quality assurance protocols in large-scale production can also lead to batch-to-batch variability. Establishing clear guidelines and standards for production and characterization is necessary to ensure quality and reliability for larger applications.
Future Directions in Research
Research in electrospinning scaffolds is rapidly evolving, and understanding future directions is vital for pushing the boundaries of tissue engineering and regenerative medicine. These scaffolds offer unique properties that can promote cellular functions, making them suitable for various medical applications. However, ongoing research will determine how effectively these structures can be integrated into clinical practices.
Integration with Other Technologies
One promising area of research is the integration of electrospinning technology with other advanced techniques. This includes combining electrospinning with 3D bioprinting, which allows for the creation of complex tissue structures. By synchronizing the methodologies, researchers can enhance the precision in scaffold fabrication. Moreover, artificial intelligence is also being considered to optimize the electrospinning process. This could lead to tailored scaffold designs based on specific patient needs, thereby increasing efficacy and functionality.
Other technologies such as nanotechnology and microfabrication can also play an important role. For instance, incorporating nanoscale features into electrospun scaffolds may enhance their surface area, promoting better cell adhesion and proliferation. This will greatly influence the design of scaffolds aimed at specific applications, such as wound healing or drug delivery systems.
Regulatory Considerations
As research progresses, so does the necessity to address regulatory considerations surrounding electrospinning scaffolds. The pathway for these novel medical devices is complex and requires a thorough understanding of safety and efficacy. Regulatory bodies, such as the FDA in the United States, have stringent guidelines that must be followed. This covers not just the materials used in fabricating the scaffolds but also their performance in medical applications.
Navigating these frameworks is essential for researchers aiming to bring their innovations to market. It is crucial to collect comprehensive data demonstrating the biocompatibility and mechanical stability of electrospun structures. This information can influence the approval process. Therefore, understanding the regulatory landscape will guide future research endeavors and applications of electrospinning scaffolds in clinics.
To sum up, integrating electrospinning technology with other advanced methodologies and adhering to regulatory frameworks are essential for future advancements in this field. Both elements play a critical role in achieving the goal of making electrospun scaffolds a staple in modern biomedical applications.
The End
The conclusion serves a pivotal role in encapsulating the principal findings and insights presented in the article about electrospinning scaffolds. It aims to synthesize key points, emphasizing how electrospinning technology is shaping the future of biomedical applications.
In reviewing the essential characteristics of electrospinning and its advancements, the conclusion highlights how electrospun scaffolds have emerged as potent tools in tissue engineering and regenerative medicine. Their versatility in fabrication, alongside the ability to tailor mechanical and biological properties, positions them at the forefront of research. The discussion also encompasses the challenges encountered, such as scalability and stability, which are critical for translating laboratory successes into clinical realities.
"Understanding the dynamics of electrospinning scaffolds not only offers insights into their current applications but also points towards future innovations in biomedical fields."
Summary of Findings
This article has detailed the diverse aspects of electrospinning scaffolds, such as their underlying principles, the various materials involved in their creation, and multiple techniques employed in their fabrication. Importantly, the finished products' characterization has been addressed, illuminating the relationship between their morphological features and biological outcomes. Discovering how these scaffolds support cellular processes proves vital not just in tissue regeneration but also in drug delivery systems and wound healing applications.
The findings emphasize that electrospinning is not merely a technical process but a gateway to developing substantial biomedical advances. The materials selected—ranging from natural to synthetic polymers—determine key properties of scaffolds. These findings provide a clear understanding of how different structures can lead to varied performance in biological settings.
Implications for the Future
As the field of electrospinning scaffolds continues to develop, the implications for future studies and applications are multi-faceted. The integration of novel materials with enhanced functionalities, such as biodegradable composites and smart materials, suggests a promising pathway for advancements. Furthermore, understanding regulatory considerations associated with these materials presents an essential avenue for researchers moving forward.
Future research could focus on more efficient scaling-up processes. This practice would bridge the gap between bench-scale synthesis and industrial-scale production, addressing the current limitations around scalability. Additionally, the continued exploration of electrospinning's potential integrates with other technologies, such as 3D printing or bioprinting, showcasing the potential for innovation in scaffold design and application.